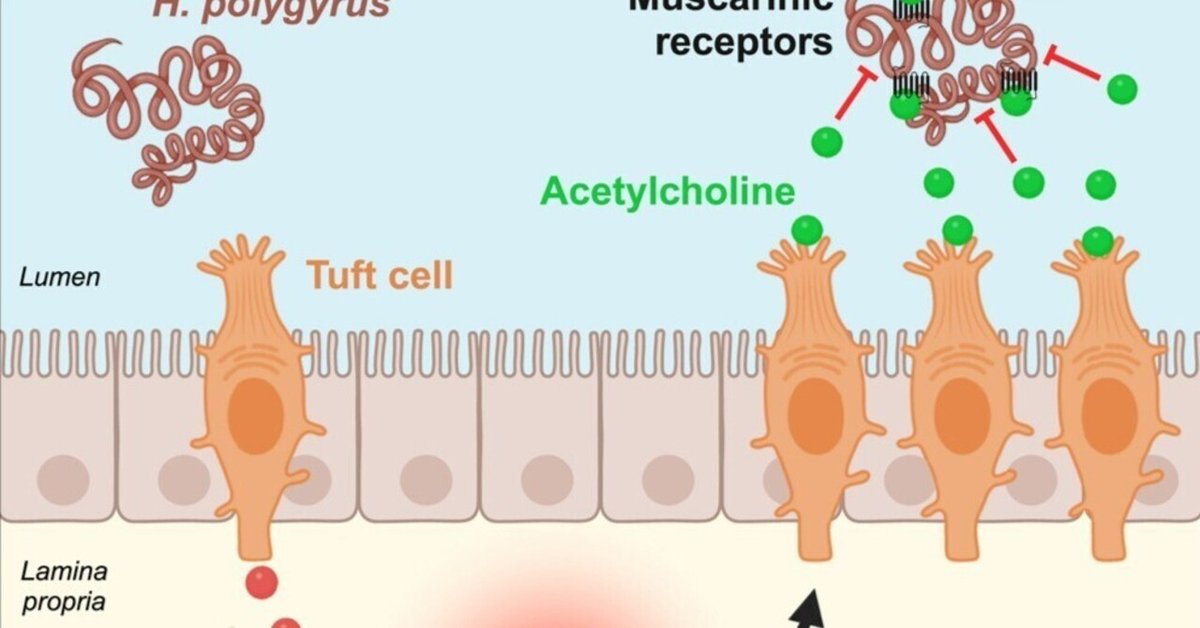
房細胞アセチルコリンは腸管内腔に放出され、抗蠕虫免疫を促進する
メインコンテンツへスキップメインメニューへスキップ
免責事項
ログイン
メインメニュー
検索...
論文|第57巻第6号、p1260-1273.e7、2024年6月11日
全号ダウンロード
房細胞アセチルコリンは腸管内腔に放出され、抗蠕虫免疫を促進する
https://www.cell.com/immunity/fulltext/S1074-7613%2824%2900223-1
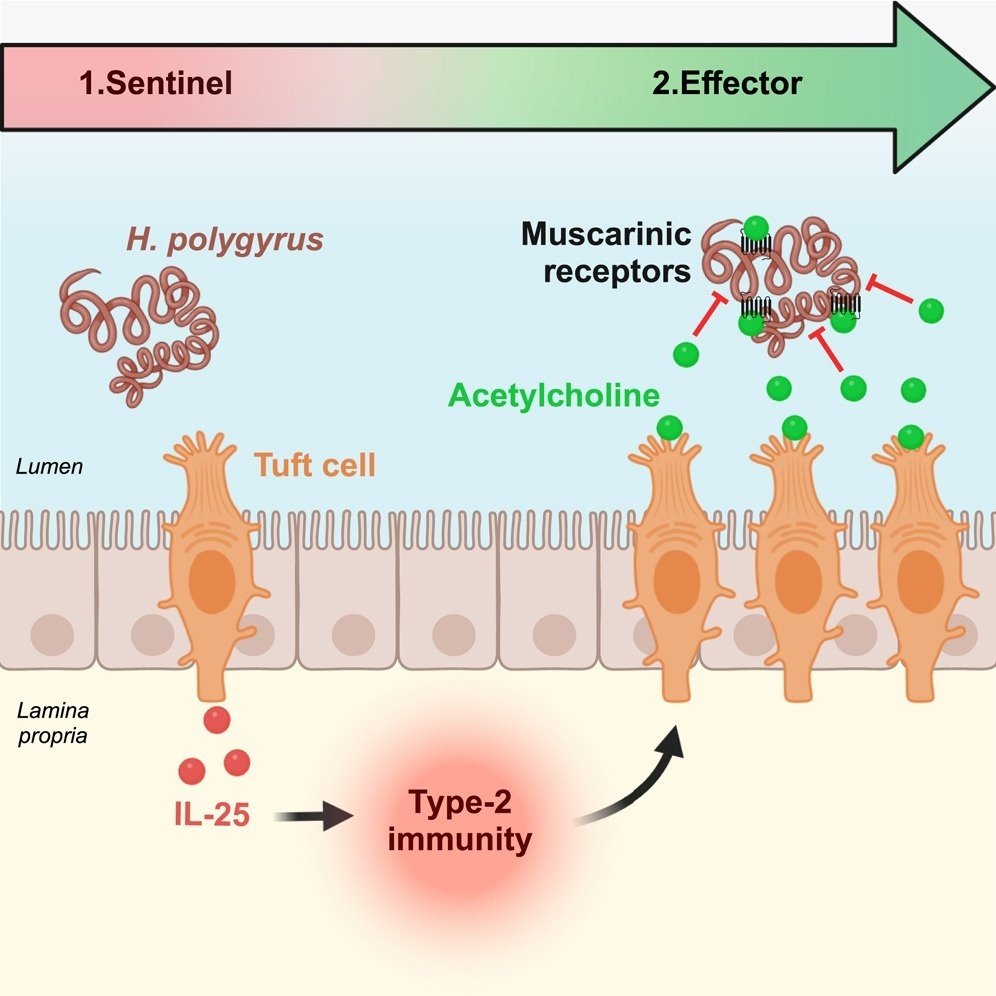
マリエーム・ンジム 9
イメーヌ・ガスミ 9
ファビアン・ヘルベール 9
リック・M・メイゼルス
フランソワ・ジェルベ
フィリップ・ジェイ 10
すべての著者を表示
脚注を表示オープンアクセス掲載:2024年5月13日DOI:https://doi.org/10.1016/j.immuni.2024.04.018
PlumXメトリクス
ハイライト
タフト細胞は2型免疫応答においてセンチネルとエフェクターの両方の役割を果たす
タフト細胞のアセチルコリン産生は蠕虫感染時に増加する。
タフト細胞は2型免疫応答時に腸管内腔にアセチルコリンを放出する。
アセチルコリンは虫のムスカリン受容体を介して蠕虫の繁殖を阻害する。
まとめ
寄生蠕虫に感染すると、活性化された腸房細胞はインターロイキン-25(IL-25)を分泌し、このインターロイキン-25が2型免疫応答を開始し、その間にlamina propriaの2型自然リンパ球(ILC2)がIL-13を産生する。これにより、房細胞過形成を含む上皮リモデリングが起こるが、その機能は不明である。我々は、コリンアセチルトランスフェラーゼ(ChAT)を発現する唯一の上皮細胞である房細胞のコリン作動性エフェクター機能を同定した。寄生虫感染時、ChATを上皮特異的に欠失させたマウスは、2型免疫応答は同等であったにもかかわらず、虫の負担、体力、糞便卵数が増加した。メカニズム的には、IL-13で増幅された房細胞はアセチルコリン(ACh)を腸管内腔に放出する。最後に、AChは蠕虫に直接作用し、蠕虫が発現するムスカリン性AChレセプターを介して、虫の繁殖力を低下させることが示された。このように、タフト細胞はナイーブなマウスではセンチネルであり、蠕虫感染時に増幅されることで、さらに2型免疫応答のエフェクター機能を提供する。
図解抄録
図サムネイルfx1
大きな画像を見るダウンロード 高解像度画像
キーワード
タフト細胞
アセチルコリン
2型免疫応答
蠕虫寄生虫
肥満細胞
自然免疫
腸管上皮
粘膜免疫
はじめに
タフト細胞は主に消化器や呼吸器の上皮に見られる細胞サブセットで、粘膜の宿主防御において重要な役割を果たしている。腸管上皮では、タフト細胞は主に寄生虫感染時の重要な見張り役として知られている。腸内に蠕虫や原虫が存在すると、タフト細胞からアラーミン・サイトカインであるインターロイキン-25(IL-25)が分泌され、2型免疫応答が開始される1,2,3。このような応答は、主にIL-4、IL-5、IL-13などの2型サイトカインの分泌を介して、2型自然リンパ球(ILC2)によって組織化される。IL-4とIL-13の機能の重要な側面は、それらが腸管上皮に引き起こす重大なリモデリングである。これには、粘液を産生する杯細胞や房細胞系列の増幅や、通常は存在しない小腸杯細胞によるレジスチン様β(Retnlβ)の異所性発現が含まれる4。Retnlβは虫の生理を直接阻害するが4,5,6、「weep and sweep」反応としても知られる粘液産生の増加と平滑筋の過収縮は虫の排出を促進する7。一方、2型免疫反応における房細胞数の劇的な増加の生理的役割はまだ解明されていない。房細胞系列の増幅に伴うIL-25産生の増加は、より効率的な2型免疫応答につながると考えられている1,3が、房細胞の別の機能も考慮する必要がある。第一に、この増幅は上皮細胞に対する2型サイトカインの作用の下流で起こったこと、第二に、IL-25欠損のみの場合と比較して、タフト細胞1がない場合は虫の排出が大幅に遅れることがわかった。これらの点から、タフト細胞はその警報機能に加え8、2型免疫反応のイニシエーターとしてだけでなく、むしろ不可欠なエフェクター成分として必要とされていることが強く示唆される。
コリンアセチルトランスフェラーゼ(ChAT)酵素によって生合成されるアセチルコリン(ACh)神経伝達物質は、神経細胞および非神経細胞のさまざまな生理機能を制御している9。Chatレポーターマウスを用いた研究から、気管、10尿道、11消化管、胆道、12胸腺など、さまざまな組織にコリン作動性上皮細胞が存在することが明らかになった13。これらの細胞は気道ではタフト(ブラシまたは孤発性化学感覚とも呼ばれる)細胞として同定され、実際のACh放出が証明された14。鼻腔では、コリン作動性タフト細胞は、刺激物質が存在する場合の呼吸と炎症を制御している15。気管では、呼吸反射16と細菌の定足数感知分子に反応する粘液毛様体クリアランスを制御している17。さらに、尿道タフト細胞は、外因性苦味化合物に反応する粘液感覚ニューロンへのコリン作動性シグナル伝達を通じて、排尿反射を制御している11。
興味深いことに、レバミゾールやピランテルなど、腸内寄生虫の駆除によく使われる薬剤はコリン作動性アゴニストである。これらは寄生虫のACh受容体(AChR)を標的とし、痙攣性麻痺を引き起こすため、寄生虫の排出が促進され18、宿主のAChが寄生虫に直接作用する可能性を示唆している。さらに、シグナル伝達に利用可能なAChは、ChAT酵素による合成とアセチルコリンエステラーゼ(AChE)酵素による分解のバランスによって生じる。粘膜表面に寄生する寄生性線虫の中には、宿主のAChへの有害な曝露を避けるために、特定の分泌腺で産生され、虫体内環境に分泌されるAChEアイソフォームをさらにコードしているものもいる19。
ここでわれわれは、2型免疫応答における房細胞由来AChの特異的な役割を調べた。タフト細胞は、ACh生合成の鍵となるChat遺伝子を発現している唯一の腸管上皮細胞であり、実際にAChを合成していることを確認した。Chat欠損の腸管上皮細胞を持つマウスでは、強力な2型免疫応答が確立されているにもかかわらず、虫の駆除が遅れていた。生体外では、AChがミミズに直接作用し、ムスカリンAChR依存的な経路で繁殖力が低下すること、またChat欠損上皮細胞を持つマウスでは一過性に肥満細胞症が低下することが示された。
研究結果
ChAT酵素をコードする遺伝子は腸上皮房細胞で特異的に発現している
小腸上皮房細胞が神経伝達物質AChを生合成する特異的な可能性を確認するため、AChの生合成を触媒するコリンアセチルトランスフェラーゼ(ChAT)酵素をコードする遺伝子の発現を、蛍光活性化セルソーティング(FACS)で濃縮した小腸EpCam+; Siglec-F+房細胞と、EpCam+; Siglec-F-非房上皮細胞画分とで比較評価した。房細胞および非房細胞画分における房細胞マーカーTrpm5 mRNAの発現を分析することにより、細胞選別法の効率を評価した。タフト細胞画分ではTrpm5 mRNAが検出されたが、非タフト細胞を含む画分では検出の閾値以下であった(図1A)。同様に、Chat mRNAはタフト細胞画分に検出されたが、小腸上皮のすべての非タフト細胞上皮サブセット(EpCam+;Siglec-F-)を含む画分には検出されなかったことから、in vivoにおける腸上皮細胞のうちタフト細胞にChat遺伝子が特異的に発現していることが示された(図1B)。次に、Chat mRNAの発現が小腸房細胞に共通した性質なのか、それともこれらの細胞のサブセットだけに限定されたものなのかを、無傷の組織という状況下で評価するために、我々はナイーブなC57BL/6マウスのChat発現細胞を可視化するために、房細胞集団全体の抗ダブルコルチン様キナーゼ1(Dclk1)免疫蛍光検出とin situハイブリダイゼーションを結合させた。この結果、Dclk1発現房細胞の大部分でChatプローブシグナルが特異的であることが明らかになった(図1C)。そこで我々は、蠕虫寄生虫感染と進行中の2型免疫応答との関連において、Chatの発現が増幅された房細胞集団にも共通する特徴であるかどうかを検討した1,3。こうして野生型マウスにHeligmosomoides polygyrus感染L3幼虫を経口投与して感染させた。そこで2回の脱皮を経て、感染後10日(dpi)頃に成虫として腸管内腔に再び出現し、そこで交尾して卵を産み、糞便中に排出される。また、Chat mRNAの発現はH. polygyrusに感染したマウスのタフト細胞に限定されており(図1D)、これは2型免疫応答においてタフト細胞以外でのde novo Chat発現がないことを示し、ほぼすべてのタフト細胞で検出された。さらに定量的比較から、ナイーブマウス(574/614個)と感染マウス(435/500個)では、Chat+ Dclk1を発現する房細胞の割合が同程度であることが明らかになった(図1E)。このように、Chat mRNA発現はマウス小腸上皮のタフト細胞に特異的に存在し、感染状態にかかわらずほぼすべてのタフト細胞で検出された。
図サムネイルgr1
図1Chat遺伝子の発現は、ナイーブマウスおよびH. polygyrus感染時の腸管上皮の房細胞に限定される
キャプション
大きな画像を見るダウンロード 高解像度画像
ChAT欠損マウスでは蠕虫寄生虫に対する宿主防御機能が低下している。
次に、in vivoの2型免疫応答における房細胞産生AChの機能を調べた。ChatLoxP/LoxP22マウスとVillin-CreERT2マウスを交配し、タモキシフェン誘導性で腸上皮細胞にCreリコンビナーゼを発現させることで、腸上皮に特異的にChat遺伝子の誘導性欠失マウスを作製した23。Chat遺伝子座におけるVillin-CreERT2を介した組換えの効率を評価するため、タモキシフェン投与ChatLoxP/LoxPマウスとChatLoxP/LoxP;Villin-CreERT2マウスにおいて、Chat遺伝子のLoxP-flanked exon 8の塩基配列をPCR法で増幅した。すべてのマウスにタモキシフェンを5日間毎日投与し、5週間後に解析した(図2A)。Chat Exon 8配列は、ChatLoxP/LoxPマウス濃縮上皮細胞のゲノムDNAで増幅されたが、ChatLoxP/LoxP;Villin-CreERT2マウスでは検出されなかった。Villin-CreERT2配列の同時増幅により、ChatLoxP/LoxP;Villin-CreERT2マウスでは、ChatLoxP/LoxPマウスと比較して、Villin-CreERT2導入遺伝子が特異的に存在すること、およびChatLoxP/LoxP;Villin-CreERT2マウスから精製されたゲノムDNAの完全性が確認された(図2B)。このことは、Villin-CreERT2導入遺伝子によるChat遺伝子座の高効率組換えを示している。さらに、Villin遺伝子プロモーターは幹細胞を含むすべての腸上皮細胞で活性であることから23、タモキシフェンによるCreERT2の活性化は、ChatLoxP/LoxP;Villin-CreERT2複合マウスの腸上皮において永久的な遺伝子欠失を引き起こす。実際、エクソン8欠失は、タモキシフェン投与5週間後にChatLoxP/LoxP;Villin-CreERT2マウス腸上皮細胞ゲノムDNAからエクソン8配列増幅が見られないことからわかるように、腸上皮細胞の急速な更新にもかかわらず、非常に安定していた(図2Aおよび2B)。なお、Villin-1とChatの発現は、小腸2型自然リンパ球(ILC2)とILC3免疫細胞集団のサブセットで報告されている(Immunological Genome Project [ImmGen]データベース)。ChatLoxP/LoxP;Villin-CreERT2マウスの解析において、上皮以外のCreERT2の関与を除外するために、Villin-CreERT2導入遺伝子産物の発現、より正確には、タモキシフェン投与による核内転座を、小腸固有層ILC1、ILC2、およびILC3サブセットで調べた。タモキシフェン注射後(図 2C)、核内 CreERT2 はすべての上皮細胞で検出されたが、CD45+ 免疫細胞、より詳細には Tbx21+、Gata3+、および Rorγt+免疫サブセットでは検出されず(図 2D)、マウス ILC1、ILC2、および ILC3 サブセットに Villin-CreERT2 導入遺伝子が発現していないことが確認された。
図サムネイルgr2
図2Villin-CreERT2導入遺伝子によって駆動される永続的かつ腸上皮細胞制限的組換え
キャプション
大きな画像を見るダウンロード 高解像度画像
次に、上皮チャットの欠損が腸粘膜の細胞組成に及ぼす影響を調べた。ChatLoxP/LoxP;Villin-CreERT2マウスにタモキシフェンを投与しても、ChatLoxP/LoxPコントロールマウスと比較して、上皮層の肉眼的変化は認められなかった。さらに、腸の造血細胞コンパートメント(上皮内および固有層分画)内の免疫サブセット(T細胞、B細胞、および単球、好中球、マクロファージ、肥満細胞を含む骨髄系細胞集団)の全体的な分布と、より遠くの末梢リンパ系臓器(脾臓、腸間膜、腋窩/上腕/頸部リンパ節)の分布は、ChatLoxP/LoxP間で同等であった; Villin-CreERT2マウスとChatLoxP/LoxPマウスの間で同等であった(図S1BおよびS1C)。より具体的には、CD4およびCD8 T細胞サブポピュレーション、ならびにそれらの活性化および極性化状態に差は認められなかった(図S1B)。同様に、ChatLoxP/LoxP;Villin-CreERT2マウスとChatLoxP/LoxPマウスの前膜および上皮内集団では、ILC1、ILC2、ILC3サブセットの相対的割合は同等であった(図S1C)。次に、寄生蠕虫感染時のChAT欠損の影響を評価した。まず、5日間連続でタモキシフェンを投与してChat遺伝子の欠失を誘導した。5日間タモキシフェンを投与せずに休息させた後、ChatLoxP/LoxPマウスおよびChatLoxP/LoxP;Villin-CreERT2マウスをH. polygyrus L3に経口感染させ、腸管内腔に成虫が存在する感染後10日から40日までの感染パラメータを解析した(図3A)。ChAT欠損マウスは明らかな表現型を示さなかったが、剖検ではChatLoxP/LoxP対照マウスと比較して成虫数が増加していた(図3B)。生きた虫の数の増加は、感染マウスの糞便中の卵の数にも反映され、2型免疫応答の効率と虫の持続性を動的に読み取ることができた。実際、ChatLoxP/LoxPの同胞と比較して、ChAT欠損マウスでは感染後10日目(成虫が腸管内腔に存在し始める頃)から感染後40日目にかけて、卵の数が有意に増加していた(図3C)。こうして、ChAT欠損マウスにおける成虫数と糞便卵数の増加は、蠕虫寄生虫に対する効率的な防御に房細胞由来のAChが不可欠な役割を果たすことを明らかにした。
図サムネイルgr3
図3Tuft細胞Chat欠損は虫の排出を遅らせる
キャプション
大きな画像を見るダウンロード 高解像度画像
ACh濃度は2型免疫応答時にタフト細胞で増加する。
次に、FACSで選別した上皮性房細胞画分の溶解液を用いて、質量分析により房細胞中の実際のACh濃度を評価した。ACh濃度は、H. polygyrusに感染したマウスのEpCam+; Siglec-F+房細胞画分では、未感染マウスの房細胞画分と比較して有意に上昇していたが、感染マウスではACh濃度のばらつきが大きく、これは感染効率の違いを反映していると考えられた(図3D)。この上昇は房細胞に富む細胞集団で起こったことから、2型免疫反応に伴う上皮のリモデリングによって房細胞数が増加した結果というよりも、効率的に感染したマウスの房細胞あたりのACh合成の増加を反映している可能性が高い。予想通り、AChはChatLoxP/LoxP;Villin-CreERT2マウスのChat欠損上皮細胞の房細胞画分からは検出されなかった(図3D)。このように、H. polygyrus感染に対する2型免疫応答において、基礎的な房細胞ACh生合成率は有意に増加した。
ChAT欠損マウスはタイプ2の免疫応答を行うことができる。
ChatLoxP/LoxP;Villin-CreERT2マウスで寄生虫クリアランスが低下するメカニズムを理解するために、未感染マウスとH. polygyrus感染マウスで、2型免疫応答とその後の上皮リモデリングの重要なパラメーターを評価した。Gata3+ILC2s/Tヘルパー2(Th2)細胞数、上皮房細胞数、杯細胞数などの2型免疫応答、小腸杯細胞によるRetnlβペプチドの発現を定量化したところ、ChatLoxP/LoxPコントロールマウスとChatLoxP/LoxP; Villin-CreERT2マウスでは、これらのパラメーターのいくつかは、感染したACh欠損マウスでさらに高く、おそらくより多くのワムシが存在するためであろう(図4A-4C )。感染したChAT欠損マウスで2型免疫パラメータが増加したのは、虫の負担が大きかったためなのか、それともChAT欠損が直接の原因なのかを調べるため、ChatLoxP/LoxPマウスとChatLoxP/LoxP;Villin-CreERT2マウスをコハク酸で処理することで、虫に依存しない房細胞の活性化とそれに続く2型免疫応答を引き起こした。コハク酸は、コハク酸受容体1(Sucnr1)を発現する唯一の腸管上皮細胞であるタフト細胞と、それに続く2型免疫応答の活性化因子として知られている。このことから、H. polygyrus感染ChatLoxP/LoxP;Villin-CreERT2マウスにおける2型免疫パラメータの増加は、ChAT欠損の直接的な影響というよりも、虫体負担の増加による可能性が高いことが示唆された。また、ChatLoxP/LoxPおよびChatLoxP/LoxPから単離した腸管上皮細胞抽出物を用いて、2型免疫応答の房細胞メディエーターおよびACh合成・輸送に関与することが知られている因子(Alox5、Alox5ap、Ltc4s、Ptgs1、Ptgs2、Hpgds、Pou2f3、Dclk1、Sucnr1、Il25、Chat、VAChT遺伝子)のmRNA発現を評価した; Villin-CreERT2から単離した腸管上皮細胞抽出物を用いた。polygyrusに感染させた。予想通り、Chat mRNAはChatLoxP/LoxP;Villin-CreERT2マウスの抽出液には存在しなかった。感染後40日目のChatLoxP/LoxP;Villin-CreERT2マウスで上昇したDclk1 mRNAを除いて、ナイーブな状態でも感染した状態でも、コントロールマウスとChAT欠損マウスの間に有意差は認められなかった(図S3)。このことは、抗体またはプローブが入手できたすべてのマーカー(Alox5ap、Alox5、Ltc4s、Ptgs2、Hpgds、Sucnr1)について、免疫蛍光法またはin situハイブリダイゼーションを用いて、組織切片の細胞スケールで確認した。ここでも、これらのマーカーの発現パターンに変化は検出されなかった。このことは、ChAT欠損が、房細胞アルマリン分子の発現やその生合成経路を実質的に変化させないことを示唆している(図S4)。
図サムネイルgr4
図4Chat遺伝子欠損は2型免疫応答の確立を阻害しない
キャプション
大きな画像を見るダウンロード 高解像度画像
これらのデータを補完するために、コントロールマウスとChAT欠損感染マウスの腸粘膜と遠隔リンパ系臓器に存在する主要な免疫細胞集団の分布も評価しようとした。残念ながら、感染マウスの炎症腸粘膜から解離した細胞の生存率が低かったため、このような解析はできなかった。しかし、遠くの末梢リンパ臓器(腸間膜リンパ節、腋窩/上腕/頚部リンパ節、脾臓)の免疫表現型解析は可能であり、ChAT欠損感染マウスではコントロールと比較して有意差は検出されなかった(図S5およびSTAR Methods)。このことから、房細胞ACh欠損の影響は局所にとどまり、遠隔または局所のリンパ系構造には影響しないことが示唆された。
マスト細胞は蠕虫寄生虫に対する防御に関与するもうひとつの免疫サブセットを構成しており26、感染マウスから解離した細胞を研究する難しさを回避するために、炎症組織切片の免疫組織化学によって分析することができる。マスト細胞プロテアーゼ1(Mcpt1)マーカーを用いて、ナイーブマウスと感染マウスのマスト細胞の存在を定量化した。ChatLoxP/LoxP;Villin-CreERT2マウスでは、Mcpt1+細胞集団はコントロールと比較してより不均一であった。そこで、コントロールマウスとChAT欠損マウスにおける肥満細胞集団の違いを定量化するために、感染マウスで検出された肥満細胞集団を、肥満細胞の密度が高い腸管組織の割合と、肥満細胞の密度が高い領域における顕微鏡視野あたりの肥満細胞数の両方で特徴付けた(動画S1、S2、S3、S4、S5、S6)。ChatLoxP/LoxPマウスではまれであったMcpt1+細胞は、H. polygyrus感染マウスでは感染20日後あるいは40日後に強く増加した。ChAT欠損マウスでは、Mcpt1+細胞数も感染後20日目に増加したが、コントロールマウスに比べるとその程度は小さく、感染後40日目では、ChatLoxP/LoxPマウスとChatLoxP/LoxP;Villin-CreERT2マウスの両方で、Mcpt1+肥満細胞数が同様に増加していた(図5A-5C )。両遺伝子型において、Mcpt1+肥満細胞は、CD63だけでなく、CD117(主にcKitやGranzyme B [GzmB]と呼ばれる)などの他の肥満細胞マーカーをほぼ常に共発現しており、活性化状態を示唆していた(図5DおよびS6A)。さらに、CD63を共発現するMcpt1+細胞の定量化により、ChatLoxP/LoxPマウスとChatLoxP/LoxP;Villin-CreERT2マウスの肥満細胞活性化率は同程度であり、20dpiではそれぞれ92.9%と97.3%、40dpiでは91.2%と92.4%であった(図S6B)。これらのデータを総合すると、ChatLoxP/LoxP;Villin-CreERT2 ACh欠損マウスは、房細胞由来のAChがない場合でも、マスト細胞を除いて強力な2型免疫応答を行うことができたが、ChAT欠損マウスでは、感染後20日目にマスト細胞の数が一過性に減少した。従って、ChAT欠損マウスにおける高い虫の持続性と卵産生は、グローバルに低下した2型免疫応答によるものではなく、虫の排出を促進するAChの未知の役割を示すものであった。
図のサムネイルgr5
図5H.polygyrus感染後、Tuft細胞に制限されたChat遺伝子欠損は腸管肥満細胞症の確立を阻害する。
キャプション
大きな画像を見るダウンロード 高解像度画像
.mp4をダウンロード (7.22 MB)
.mp4ファイルのヘルプ
ビデオ S1. H.ポリ感染20日後のコントロールマウス(ChatLoxP/LoxP)の全腸管ロールの代表的スキャン(Granzyme-B(緑)とDAPI(青)で染色)。
ダウンロード.mp4 (6.8 MB)
.mp4ファイルのヘルプ
ビデオS2. H.ポリ感染20日後のChatタフト細胞欠損マウス(ChatLoxP/LoxP;Villin-CreERT2)の全腸管ロールの代表的スキャン(Granzyme-B(緑)およびDAPI(青)で染色)。
ダウンロード.mp4 (7.52 MB)
.mp4ファイルのヘルプ
ビデオS3. H.ポリ感染40日後のコントロールマウス(ChatLoxP/LoxP)の全腸管ロールの代表的スキャン(Granzyme-B(緑)およびDAPI(青)で染色)。
.mp4をダウンロード (6.09 MB)
.mp4ファイルのヘルプ
ビデオS4. H.ポリ感染40日後のChatタフト細胞欠損マウス(ChatLoxP/LoxP;Villin-CreERT2)の全腸管ロールの代表的スキャン(Granzyme-B(緑)およびDAPI(青)で染色)。
ダウンロード.mp4 (7 MB)
.mp4ファイルのヘルプ
ビデオS5. ナイーブコントロールマウス(ChatLoxP/LoxP)の全腸管ロールの代表的スキャン(Granzyme-B(緑)とDAPI(青)で染色)。
ダウンロード.mp4 (6.87 MB)
.mp4ファイルのヘルプ
ビデオS6. ナイーブなChat tuft細胞欠損マウス(ChatLoxP/LoxP;Villin-CreERT2)の腸管ロール全体の代表的スキャン(Granzyme-B(緑)とDAPI(青)で染色)。
H.polygyrus感染後、管腔ACh濃度が上昇する。
ある種の蠕虫寄生虫はAChE酵素の分泌型アイソフォームを発現しており27 、近隣のAChを分解することが虫にとって有益であることを示唆している。房細胞由来のAChが宿主の腸管内腔に存在するワムシに直接作用するという仮説を検討するため、次に、未感染マウスとH. polygyrus感染マウスで腸管内腔ACh濃度を評価した。このために、腸管ループを外科的に結紮し、洗浄液で満たし、30分間のインキュベーション後に回収し、質量分析によるACh検出のために処理した。その結果、コントロール感染マウスの腸管内腔におけるACh濃度が、ナイーブ動物と比較して有意に上昇していることが明らかになった(図6A)。これは、FACSで選別したタフト細胞の抽出物からAChを定量した結果、タフト細胞数が多いことと、タフト細胞あたりのACh産生が増加していることの両方が寄与していると考えられる。感染マウスのACh濃度は様々であったが、これはおそらく感染効率と、手術に適した腸管ループに関する虫の特異的な位置を反映していると思われる。次に、管腔ACh濃度の上昇には、寄生虫の実際の存在が必要なのか、それともこの上昇が房細胞の活性化に直接関係しているのかを検討した。そこでマウスをコハク酸で処理し、ACh濃度の変化を評価した。実際、コハク酸処理した野生型マウスの腸管内腔では、未処理のマウスと比較して、内腔AChも有意に増加したことから、虫がいない状態で活性化された房細胞による内腔ACh放出が示唆された(図6B)。これらのデータを総合すると、H. polygyrus感染マウスの腸管内腔に房細胞由来のAChが存在することが証明され、内腔AChが虫の生理機能に直接影響している可能性が示唆された。
図サムネイルgr6
図6腸房細胞由来のAChが宿主の腸管内腔に放出され、AChが虫の生理機能を直接障害している。
キャプション
大きな画像を見るダウンロード 高解像度画像
ACh欠損マウスにおけるワムシの体力増加
H.polygyrusがAChに暴露された結果を直接評価するために、2つの相補的なアプローチを用いた。注目すべきことに、腸杯細胞はRetnlβ分子を産生し、特に2型免疫応答との関連で、この分子はH. polygyrusの生理を直接阻害する4。その後、Retnlβに曝露されたワムシはATP濃度が低下し、曝露されていないワムシと比較して生存率と繁殖力が変化することがわかった5,6。そこで、同様のアプローチを用いて、ChatLoxP/LoxPマウスとChatLoxP/LoxP;Villin-CreERT2マウスの腸から回収したワムシのATP濃度を、グローバルフィットネスの代用として定量した。感染後20日目に回収された両遺伝子型マウスのミミズのATP濃度に有意差は認められなかった。対照的に、40日目までに、ACh欠損房細胞を持つマウスのワムシでは、コントロールのChatLoxP/LoxP同腹体と比較して、有意にATP濃度の上昇が認められ、数週間AChを含む環境から得られたワムシのフィットネスが低下していることが示唆された(図6C)。
ACh暴露はワムシの繁殖力を直接低下させる
この所見を確認するため、10μM ACh存在下でのワムシの繁殖力も評価した。これは、H. polygyrus感染ChatLoxP/LoxP対照マウスから得られた腸管内腔洗浄液中のマイクロモル領域のACh濃度と一致する。なお、30分間の洗浄で腸管内AChが部分的に回復することは確かであり、腸管ループに注入された洗浄液量に相当する希釈倍率は15倍と推定される(図S7)。このようにして、感染後14日目にChatLoxP/LoxPマウスから回収したばかりのH. polygyrus成虫雌虫1匹あたりの卵産生量を定量し、虫が腸管内腔に存在し、内腔AChによってまだ強く変化していないことを確認した。AChを培養液に添加すると、卵の産生は未処理のワムシと比較して有意に減少した(図6D)。これらのデータは、生理学的に適切な濃度のAChにワムシがさらされると、蠕虫の繁殖力が直接低下することを示している。
AChはムスカリン性AChレセプターを介して蠕虫の生理機能を標的とする。
AChが蠕虫の生理に及ぼす影響の根底にあるメカニズムを明らかにするため、AChとコリン作動性シグナル伝達のさまざまな阻害剤を併用した状態で、蠕虫の繁殖力5,6を評価した。図6Dに示したように、AChを単独で投与すると、未処置のワムシと比較して、ワムシの繁殖力が低下した。AChとニコチン性AChRsのアンタゴニストであるメカミルアミンを併用すると、ミミズの繁殖力は同程度に有意に低下した。対照的に、AChとムスカリンAChRsのアンタゴニストであるアトロピンを併用したワムシでは、AChによる産卵阻害効果は観察されなかった(図6E)。このことから、AChの産卵阻害作用は、ミミズの発現するムスカリン性AChRによって媒介されていることが示唆された。最後に、いずれの薬剤も単独で用いた場合には産卵に有意な影響を及ぼさなかった(図6E)。これらのデータを総合すると、腸房細胞は2型免疫応答を開始するセンチネル機能に加えて、生体外実験(図7)で評価されたように、ムスカリンAChRを介して蠕虫の繁殖力を直接変化させることができる濃度の非神経性AChを宿主内腔に放出することにより、そのような応答のエフェクターとして働くことが示唆される。さらに、H. polygyrus感染後の活性化マスト細胞の増加には、体腔内または基底側から分泌されたAChが関与している可能性が高い。
図のサムネイルgr7
図7蠕虫寄生虫感染時の房細胞エフェクター機能を示す模式図
キャプション
大きな画像を見るダウンロード 高解像度画像
考察
これまでのところ、房細胞産生AChの重要性は主に気道で研究されてきた。マウスの気管上皮では、タフト細胞は気道内膜液中に存在する苦味成分を感知する能力があり、コリン作動性シグナルを近隣の神経終末に伝達することで、呼吸回数の減少からなる嫌悪反射を引き起こす10。これらの細胞は、3-OxoC12-HLSのような、微生物が自らの集団密度を評価するために使用する細菌クオラムセンシング分子(QSM)によって活性化され、AChシグナルを用いて呼吸変化を引き起こす16。呼吸反射に加えて、粘膜繊毛クリアランスは、吸入した病原体を気道から排除するための重要な生得的防御プロセスである。気管房細胞からのパラクリン性コリン作動性シグナル伝達は、苦味化合物、QSM、その他の細菌由来産物によって活性化され、気管表面上の粒子の輸送速度によって評価されるように、気道繊毛細胞による粘膜繊毛クリアランスを増加させることが見いだされ、それによって細菌シグナルの化学感覚と自然防御が直接的に結びついている14,17。レポーターマウスを用いた以前の研究では、Chat11とTas2r143苦味受容体29が、消化管だけでなく泌尿生殖器の房細胞のサブセットでも発現していることが報告されている。Chat遺伝子座を駆動源とする緑色蛍光タンパク質(GFP)レポーターを用いた先行研究では、気管と尿道のビリン免疫反応性房細胞におけるGFP発現の不均一性が報告されている。
われわれは、小腸房細胞のコリン作動性機能について2つの側面から検討した。第一に、マストサイトーシスに対する制御因子の役割を明らかにしたが、これがH. polygyrusの動態に与える具体的な影響については、現在のところ解明が困難である。第二に、腸管内腔AChの産生を介したタフト細胞のエフェクター機能を報告した。実際、H. polygyrus感染マウスの2型免疫応答において、腸管内腔にAChが存在し、その濃度はマイクロモル領域に達することが報告された。さらに、管腔ACh濃度の上昇は、コハク酸処理による房細胞の活性化によっても引き起こされることから、管腔ACh産生は、房細胞活性化の下流にある2型免疫応答に不可欠な要素であることが示唆された。タフト細胞由来のAChは、感染後40日目のワムシの生存率を直接的または間接的に低下させたが、ワムシの全球ATPレベルで評価すると、感染後20日目には低下しなかった。さらに、AChを24時間曝露すると、H. polygyrusのムスカリンAChRを介して、ワムシの繁殖力に直接的な影響を及ぼすことが生体外で証明された。一方では、in vivoでの全ミミズATP濃度の定量が、他方では、ex vivoでの産卵が、AChに依存した異なる生理機能の変化を反映している可能性がある。そのため、宿主由来のAChが、関連するH. polygyrusのムスカリンAChR経路を直接阻害し、繁殖力を低下させている可能性が高い。感染後40日目に観察されたワムシの体力低下は、おそらくニコチン性AChRと神経筋活動や摂食などの関連機能が関与する、異なる経路の変化に依存しているのかもしれない31。
タイプ2の免疫反応の中で房細胞から放出される内腔AChは、異なる上皮サブセットがいかにこのプロセスの不可欠な部分を構成しているかを示している。上皮IL-4Rαレセプターを介したシグナル伝達は、房細胞および杯細胞という2つの上皮細胞系譜の特異的増幅を引き起こす。上述のタフト細胞のエフェクター機能に加え、杯細胞は粘液産生の増加によっていわゆるweep and sweep応答に寄与するだけでなく、蠕虫の宿主組織への摂食能力と直接相互作用するRetnlβペプチドをde novoで発現するため、蠕虫の体力と繁殖力を低下させることにも寄与する4,5,6。寄生蠕虫は宿主と共進化してきたため、AChやRetnlβのような、それぞれ房細胞や杯細胞から産生される、異なる宿主細胞型に依存する異なる防御機構を用いることで、寄生虫が宿主の免疫に対抗することがより困難になっていると考えられる。このような観点から、AChとRetnlβの宿主欠損を組み合わせて、異なる蠕虫寄生種に対するこれら2つの分子の相乗作用の可能性を調べることは興味深いかもしれない。
免疫反応のコリン作動性制御については、蠕虫感染症を含め、よく報告されている。というのも、ChatLoxP/LoxP;Villin-CreERT2マウスでは、強力な2型免疫応答にもかかわらず、房細胞由来の免疫メディエーターの発現に新たな変化は認められず、免疫集団の有意な変化も確認されなかったからである。しかしながら、ChAT欠損マウスでは、H. polygyrus感染後に観察される肥満細胞症が部分的に抑制されることが報告された。肥満細胞と2型免疫応答との正確な関連はまだ部分的にしか解明されていないが、複数の研究から、肥満細胞欠損マウス系統では、H. polygyrus感染後を含め、抗寄生虫防御能が低下することが報告されている37,38。このように、蠕虫感染後の肥満細胞症は、房細胞由来のAChがなくても起こるが、感染後20日目には、感染した対照マウスと比較して量的に低下しており、ChatLoxP/LoxP;Villin-CreERT2マウスで観察されたH. polygyrus感染制御の遅れの一因となっている可能性がある。注目すべきことに、蠕虫感染における肥満細胞の役割に関するいくつかの研究では、肥満細胞の欠損を引き起こすためにcKit遺伝子の機能喪失モデル37,39,40やその薬理学的阻害38,41が用いられている。cKitは造血前駆細胞42や上皮性のパネス細胞43、杯細胞44、房細胞45にも発現しており、肥満細胞の欠損と並行して房細胞の欠損が誘導された場合、混乱が生じる可能性がある。
小腸における房細胞AChの作用様式は、AChの管腔放出と粘膜マストサイトーシスの微調整だけではないかもしれない。気道で報告されたように、他の上皮細胞や神経細胞へのパラクリンシグナル伝達など、さらなるコリン作動性メカニズムが、最近小腸で報告された。
このように、腸房細胞のコリン作動性機能に関する我々の研究により、AChの管腔内作用様式を持つ腸房細胞の新たな機能が明らかになった。従って、蠕虫感染に対する宿主防御において、タフト細胞は2つの異なる、しかし相補的な役割を果たしていると考えられる。ナイーブな粘膜では、タフト細胞は寄生虫感染時に2型免疫応答を開始するセンチネルとして機能するまれな上皮サブセットである。我々の発見は、現在進行中の2型免疫応答の中で起こるタフト細胞系の増幅が、マストサイトーシスのコリン作動性制御因子としての第二の機能と、寄生蠕虫のグローバル・フィットネスを低下させることによって寄生蠕虫のクリアランスに直接寄与すると思われるエフェクターとしての機能の根底にあることを明らかにした。さらに、ex vivoで実証されたように、腸管内腔で測定されたACh濃度は、H. polygyrusの繁殖力を直接変化させることができる。このことは、2型免疫応答におけるタフト細胞の過形成の役割を明らかにするだけでなく、タフト細胞のセンチネル機能に関与する重要なサイトカインであるIL-25の欠損8が、Pou2f3欠損マウスにおけるタフト細胞の完全な欠損よりも重篤な表現型を引き起こさない理由を理解するためのミッシングリンクを提供するかもしれない12。
研究の限界
これらの知見にもかかわらず、いくつかの疑問が残っている。第一に、ACh分泌の基礎となる房細胞のメカニズムはまだ不明である。シナプス小胞へのAChの取り込みに必要なVAChTタンパク質と、細胞内ACh合成を促進するためのコリンの再取り込みに必要な高親和性コリントランスポーター(ChT1)に関する以前の発現研究では、すべての腸管分節の房細胞でこれら2つのタンパク質が検出可能な発現を示さなかったことが報告されている12。第2に、本研究は、房細胞が蠕虫寄生虫を直接標的とする分子としてAChを放出するという概念を導入した。この考え方は、蠕虫や他の腸内寄生虫に対して同じ働きをする房細胞因子がさらに発見されれば、さらに広がるかもしれない。最後に、コリン作動薬やAChE阻害薬のような、ヒトや家畜の一般的な抗蠕虫治療薬を改良する上で、これらの薬剤の管腔内作用様式に焦点を当てて、我々の発見に連続する潜在的な治療的展望を評価するための今後の研究が必要であろう。
STAR★メソッド
主要リソース表
試薬またはリソースソース識別子
抗体
ウサギ ポリクローナル抗 Dclk1 Abcam Cat# Ab31704; RRID: AB_873537
Dclk1 に対するヒツジポリクローナル R&D Systems Cat# AF7138; RRID: AB_10973467
Gata3 に対するウサギのモノクローナル [EPR16651] Abcam Cat# Ab199428; RRID: AB_2819013
Retnlb に対するウサギのポリクローナル抗体 オンライン Cat# ABIN465494
Insm1 に対するマウスモノクローナル Santa Cruz Cat# sc-271408; RRID: AB_10607955
リゾチームに対するウサギのポリクローナル Dako Cat# A0099; RRID: AB_2341230
Granzyme-B 抗体に対するウサギのポリクローナル Abcam Cat# Ab4059; RRID: AB_304251
Cd45 に対するラットモノクローナル抗体 Novus Cat# NB100-77417; RRID: AB_1083776
MCPT-1 (mMCP-1) に対するラットモノクローナル抗体 eBioscience/ThermoFisher Cat# 14-5503-82; RRID: AB_10854869
C-Kit抗体に対するヤギポリクローナル R&D Systems Cat# AF1356; RRID: AB_354750
コラーゲン V に対するウサギのポリクローナル抗体 Patricia Simon Assmann からの寄贈 N/A
ラット抗マウス Siglec-F, PE-conjugated BD pharmigen Cat# 552126; RRID: AB_394341
ラット抗マウス EpCam、APC 標識 eBioscience/ThermoFisher Cat# 17-5791-82;
RRID: AB_2716944
5-リポキシゲナーゼ抗体(5Alox)に対するウサギモノクローナル Novus Cat# NBP3-161-50
ヤギ FLAP (Alox5Ap) に対するポリクローナル抗体 Novus Cat# NB300-891; RRID: AB_2227081
シクロオキシゲナーゼ 2 に対するウサギのポリクローナル抗体 Abcam Cat# Ab225273
Prostaglandin D Synthase (hematopoietic-type) に対するウサギのポリクローナル抗体 Cayman chemical Cat# 160013;
RID: AB_10080037
Rabbit monoclonal to CD63 antibody Abcam Cat# Ab217345;
RID: AB_2754982
エストロゲン受容体αに対するマウスモノクローナル抗体 Santa Cruz Cat# Sc-8002;
RID: AB_627558
ウサギ抗 TBX21(T-bet)モノクローナル Clinisciences Cat# AC-0240A
ウサギ抗 Rorgt(クローン EPR20006) Abcam Cat# ab207082; RRID: AB_2889310
ラット抗 mCD45-FITC (クローン 30-F11) BD Biosciences Cat# 553080; RRID: AB_394610
ラット抗mCD45-APC(クローン30-F11) BD Biosciences Cat# 559864; RRID: AB_398672
Rat Anti-mCD45- APC-eF780 (clone 30-F11) eBioscience/ThermoFisher Cat#47-0451-80;
RID: AB_1548790
ラット抗 mCD19- BV650 (クローン 1D3) BD Biosciences Cat# 563235;
RID: AB_273808
ラット抗mCD19- V450(クローン1D3) BD Biosciences Cat# 560375;
RID: AB_164526
ハムスター 抗 CD11c- PE (クローン HL3) BD Biosciences Cat#557401; rid: ab_164526
RID: AB_396684
ラット抗mCD11b-BV605(クローンM1/70) BD Biosciences Cat# 563015; RRID: AB_2737951
Rat Anti-mCD4- BV711 (clone RM4-5) BD Biosciences Cat#563726;
RRID: AB_2738389
Rat Anti-mCD4- BV786 (clone RM4-5) BD Biosciences Cat# 563727;
RID: AB_2728707
ハムスター抗mTCRβ- FITC(クローンH57-597) BD Biosciences Cat# 553170; RRID: AB_394682
Hamster Anti-mTCRβ- AF594 (clone H57-597) Biolegend Cat# 109238;
RRID: AB_2563324
ハムスター抗 mTCRβ- V450 (クローン H57-597) BD Biosciences Cat# 560706; RRID: AB_1727576
ラット 抗 mCD8α- APC-R700 (clone 53-6.7) BD Biosciences Cat#564983;
RRID: AB_2739032
ラット抗 mCD8b- PE (クローン H35-17.2) BD Biosciences Cat# 550798; RRID: AB_393887
ラット抗mCD117- BV711 (clone2B8) BD Biosciences Cat# 563160; RRID: AB_272251
ラット抗mCD117- PECF594 (clone2B8) BD Biosciences Cat# 562417; RRID: AB_11154233
Rat Anti-mSca-1- FITC (clone D7) BD Biosciences Cat# 557405;
RRID: AB_396688
ラット抗 mCD127- PE (クローン A7R34) eBioscience/ThermoFisher Cat# 12-1271-82; RRID: AB_465844
ハムスター抗 mKLRG1- PeCy7 (クローン 2F1) eBioscience/ThermoFisher Cat# 25-5893-82; RRID: AB_1518768
Anti-mGATA3- V450 (clone L50-823) BD Biosciences Cat# 563349;
RRID: AB_2738152
マウス抗mRoRγt- BV650 (clone Q31378) BD Biosciences Cat# 564722; RRID: AB_2738915
マウス抗 mTbet- PE (クローン 4B10) eBioscience/ThermoFisher Cat#12-5825-82;
RID: AB_925761
ラット抗 mF4/80- PeCy7 (クローン BM8) eBioscience/ThermoFisher Cat# 25-4801-82;
RID: AB_469653
ラット抗mLy6C- V450(クローン HK1.4) eBioscience/ThermoFisher Cat# 48-5932-82; RRID: AB_10805519
ラット抗 mLy6G- PE-CF594 (clone 1A8) BD Biosciences Cat# 562700; RRID: AB_2737730
Hamster Anti-mFceRIα - APC (clone MAR-1) eBioscience/ThermoFisher Cat# 17-5898-80;
RID: AB_10717073
ライブ/デッド V506 eBioscience/ThermoFisher Cat#65-0866-14
Donkey Anti-Rabbit IgG H&L (Alexa Fluor® 555) Abcam Cat# ab150074;
RID: AB_2715537
Donkey Anti-Rabbit IgG H&L (Alexa Fluor® 647) Abcam Cat# ab150075;
RID: AB_2752244
Donkey Anti-Rat IgG H&L (Alexa Fluor® 647) preadsorbed Abcam Cat# ab150155;
RID: AB_2813835
Donkey Anti-Rat IgG H&L (Alexa Fluor® 488) preadsorbed Abcam Cat# ab150153;
RID: AB_2737355
Donkey Anti-Mouse IgG H&L (Alexa Fluor® 555) Abcam Cat# ab150106;
RID: AB_2857373
Donkey Anti-Mouse IgG H&L (Alexa Fluor® 488) Abcam Cat# ab150105;
RID: AB_2732856
Donkey Anti-Sheep IgG H&L (Alexa Fluor® 488) Abcam Cat# ab150177;
RID: AB_2801320
Donkey Anti-Goat IgG H&L (Alexa Fluor® 555) Abcam Cat# ab150130;
RID: AB_2715537
化学物質、ペプチド、組換えタンパク質
臭化ネオスチグミン Sigma-Aldrich Cat# N2001
塩化アセチルコリン Sigma-Aldrich Cat# A6625
アトロピン Sigma-Aldrich Cat# A0132
塩酸メカミルアミン Sigma-Aldrich M9020
RPMI 培地 Life Technologies 社の Cat# 21875-034
DMEM 培地 ライフテクノロジー社 Cat# 21969-035
FBS シグマアルドリッチ Cat# F7524
ディスパーゼ Corning Cat# 354235
DNAse 1 シグマアルドリッチ Cat# 11284932001
7-aminoactinomycin D ライフテクノロジーズ Cat# A1310
HBSS 10X (Ca2+/Mg2+ なし) GIBCO Cat# 14185-045
Ca2+/Mg2+ 含有 HBSS 1X GIBCO Cat# 14025-050
PBS 1X(Ca2+ および Mg2+ フリー) Eurobio Cat# CS1PB01-01
LiberaseTM Roche Cat# 5401119001
EDTA Sigma-Aldrich Cat# 03690-100
DTT Sigma-Aldrich Cat# 43816-50
ベトフルラン 1000 mg/g Virbac GTIN 03597132002653
ブプレケア 0.3 mg/ml Axience GTIN 03760087151244
タモキシフェンSigma-Aldrich Cat# T5648
ラオカイン 16mg MSD GTIN:05017363520132
トリゾール試薬 サーモフィッシャー Cat# 15596026
ブプレノルフィン Sigma-Aldrich Cat#B9275
ヘマトキシリン溶液、ギル No.3 Sigma-Aldrich Cat#GHS316
SIGMAFAST™ 3,3′-ジアミノベンジジンタブレット Sigma-Aldrich Cat# D4293
N-ヒストファイン ニチレイバイオサイエンス 414151F
重要な市販アッセイ
ATPLite キット Perkin Elmer Cat# 6016943
RNeasy Micro Kit Qiagen ID: 74004
RNeasy Mini Kit Qiagen ID: 74104
Transcriptor First Strand cDNA Synthesis Kit Roche lifescience Cat# 04896866001
LightCycler® 480 SYBR Green I マスター Roche lifescience Cat# 04887352001
RNAscope™ Multiplex Fluorescent V2 Assay ACD a biotechne brand Cat# 323270
FOXP3/ 転写因子染色バッファーセット eBioscience/ThermoFisher Cat# 00-5523-00
Arcturus RiboAmp Plus キット Thermo Fisher Cat# KIT0501
実験モデル 生物/系統
マウス系統 Chattm1Mlt Lecomte ら 22 S. Berrard 提供
マウス系統 Tg(Vil1-cre/ERT2)23Syr El Marjour 他 23 S. Robine より提供
マウス系統 ChatLoxP/LoxP; VillinCreERT2/+ 本試験 N/A
Heligmosomoides polygyrus R. Maizelsより提供 R. Maizelsより提供
オリゴヌクレオチド
チャット フォワード TTTGCAGCCAGCCTCATCTC
逆方向 TATGGCCAGGAAGCCGGTAT ユーロフィン社
VAChT フォワード GGCCTCGCTACCCCACAGAA
逆行 CCCAGGCCAATAAGCAGCGG Eurofins
Pou2f3 フォワード GAGGGAATGATGAGCCCACT
リバース GTGAAGCCTAGCTTAATGCGTC Eurofins
Alox5 フォワード TCTTCCTGGCACGACTTTGCTG
リバース GCAGCCATTCAGGAACTGGTAG ユーロフィン社
Alox5ap フォワード GTTCTTTGCCCACAAGGTGGAG
逆方向 TGCAGTCCAGAGTACCACAAGG ユーロフィン社
Ptgs1 フォワード GAATGCCACCTTCATCCGAGAAG
リバース GCTCACATTGGAGAAGGACTCC ユーロフィン社
Ptgs2 フォワード TCAATGAGTACCGCAAACGC
逆順 AGGGTACAGTTCCATGACATCG ユーロフィン社
Hpgds フォワード GAATAGAACAAGCTGACTGGC
逆順 AGCCAAATCTGTGTTTTTGG ユーロフィン社
Ltc4s フォワード CCTACAGGTGATCTGCACGA
逆行 TGGCGAGGAACAGCGGAAAGTA Eurofins
Sucnr1 フォワード CCATCTCTGACTTTGCTTTCCTG
逆順 GTGTAGAGGTTGTGTGAAGCAC ユーロフィン社
Dclk1 フォワード CAGCCTGGACGAGCTGGTGG
逆行 TGACCAGTTGGGGTTCACAT Eurofins
Il25 フォワード CCTGTCAGGCAGGGGTAGTA
逆行 CCAAGAATGCAACAGCCTG Eurofins
Villinフォワード CAAGCCTGCTCGACGGCC
逆順 CGCGAACATCTTCAGGTTCT ユーロフィン社
Chat (exon8) フォワード CCCGTTTCCTTCCAGCCAA
逆順 CGCGAACATCTTCAGGTTCT ユーロフィン社
Gapdh フォワード GGAGCGAGACCCCACTAACA
逆方向 ACATACTCAGCACCGGCCTC Eurofins
Hprt フォワード GCAGTACAGCCAAAATGG
リバース GGTCCTTTTCACCAGCAAGCT Eurofins
ソフトウェアとアルゴリズム
NdpView.V2 浜松ホトニクス https://www.hamamatsu.com
Zen 青版。V3.2 ツァイス https://www.zeiss.com/microscopy/fr/produits/logiciel/zeiss-zen.html
Photoshop CS6。V13.0 Adobe https://www.adobe.com/fr/products/photoshop.html
Prism10.1 グラフパッド https://www.graphpad.com
LightCycler 480 V1.5 Roche https://lightcycler-software.software.informer.com
ImageJ. V1.8 Wayne Rasband (NIH) https://imagej.net/ij/download.html
FlowJo, LLC V9 Tree Star; Ashland, OR https://www.flowjo.com/
Biorender バイオレンダー https://www.biorender.com/
その他
RNAscope™ Probe- Mm-Chat-C2 Advanced Cell Diagnostics Cat# 408731-C2
RNAscope™ プローブ- Mm-Sucnr1-C3 Advanced Cell Diagnostics Cat# 437721-C3
RNAscope™ プローブ- Mm-Ltc4s-C3 Advanced Cell Diagnostics Cat# 1046751-C3
新しいタブで表を開く
リソースの有無
リード連絡先
リソースおよび試薬に関する詳細情報およびリクエストは、Philippe Jay (philippe.jay@igf.cnrs.fr)までご連絡ください。
材料の入手可能性
本試験で使用した安定な試薬はすべて、材料譲渡契約書を締結した主担当者から入手可能である。
データおよびコードの入手可能性
本論文で報告されたすべてのデータは、要求があれば主担当者から提供される。
本論文ではオリジナルのコードは報告しない。
本論文で報告されたデータを再分析するために必要な追加情報は、要求があれば研究責任者から入手可能である。
実験モデルと研究参加者の詳細
マウスモデル
条件付きChatLoxP/LoxP対立遺伝子(Chattm1Mlt,22)はSylvie Berrardの好意により提供され、腸上皮特異的、タモキシフェン誘導性、Villin-CreERT2マウス系統(Tg(Vil1-cre/ERT2)23Syr.23)と交配された。すべての動物実験は、ARRIVEガイドラインに従い、2022020111554986および2019031411197134 Apafis参照のもと、実験的処置のための動物の世話および使用に関するフランス教育研究省に従って実施された。オスとメスのマウスを8週齢から12週齢の間に分析した。コントロールマウスと欠損マウスのコホートは、同腹子から得た。サンプルサイズを事前に決定する統計的方法は用いず、実験は無作為化しなかった。特に断りのない限り、研究者は実験中および結果評価中の割り付けについて盲検化されていなかった。
方法の詳細
動物実験
Creを介した組換えは、1mgのタモキシフェン(シグマ社製)を毎日腹腔内注射し、5日間連続で行った。H. polygyrus感染実験では、マウスに200匹の感染性L3を経口接種した。感染パラメータは、感染後10日目から40日目までの糞便卵数、または腸内成虫数でモニターした。腸管内腔内容物のサンプルは、以下の手術手順に従って、未感染マウスまたは感染マウスから採取した。4時間の絶食後、マウスにブプレノルフィン(0.1mg/kg体重)を皮下単回投与し、30分後に3%イソフルランで麻酔した。局所リドカイン処理後、腹腔を開き、腸管ループを結紮した。この腸管ループに、5μMの臭化ネオスチグミン(Sigma)を含むPBS洗浄液を400μLまで満たした。ループの内容物は30分後に回収し、その後マウスを安楽死させた。
H. polygyrus成虫の回収とコリン作動性薬剤による治療のためのin vitro培養
感染マウスの腸管内腔から14dpiでH. polygyrus成虫を回収し、卵放出に対するコリン作動性薬剤の影響をin vivoで評価した。ワムシをペニシリン(5U/ml)/ストレプトマイシン(5μg/ml)/ゲンタマイシン(1%)を含むPBSで洗浄し、数を数え、抗生物質を含む200μLのRPMI中、37℃、5%CO2で1ウェルあたり20~25匹の雌ワムシを培養した。その後、H. polygyrus成虫を以下の薬剤で処理した:10μMの塩化アセチルコリン(Sigma, A6625)、アトロピン(Sigma, A0132)、および5μMの塩酸メカミルアミン(Sigma, M9020)。24時間後、培地を飽和NaCl溶液(50/50容量比)と混合し、卵をカウントした。グローバルなワムシフィットネス評価のため、20dpiと40dpiでマウスからワムシを回収し、PBSで洗浄した後、ATPLiteキット(6016943;PerkinElmer)を用いて、製造元の指示に従ってATP定量用の基質として直接使用した。
細胞選別実験
HBSS pH7.4(Life Technologies)中、氷冷した30mM EDTA(Sigma)中で20分間インキュベートした後、小腸から単一の腸上皮細胞を得た。次に組織を、10%FBS(シグマ)を添加したDMEM(ライフテクノロジーズ)中、100μLのディスパーゼ(コーニング)、および100μLのDNase I at 2,000 Kunitz(シグマ)で激しく振盪した。40μmメッシュで濾過後、単細胞懸濁液をフィコエリトリンラット抗マウスSiglec-F抗体(BD Pharmigen, 552126)、FITCラット抗マウスEpCam抗体(17-5791-82, ebiosciences)と4℃で30分間インキュベートし、HBSSで洗浄後、死細胞を除くために7-アミノアクチノマイシンD(Life Technologies)で染色する前に、5%FBS添加HBSS pH7.4に適当量懸濁した。Siglec-F+生細胞は、FACSAria(Becton Dickinson)、RLT溶解バッファー(Qiagen)中で直接RNA抽出、またはACh定量アッセイ用のメタノールを用いて選別した。上記のように上皮細胞画分を除去した後、残りの組織は薄層前膜細胞調製のために進められた。組織は、5%FCSを添加したRPMI1640(Life technologies)で2回洗浄した後、細切にし、RPMI1640、100μg/mLのLiberase TM(Roche)および50μg/mLのDNase I(Sigma-Aldrich)からなる溶液で、穏やかに振盪しながら37℃で30分間消化した。上清を40μmメッシュで濾過した。2000RPM、4℃で5分間の遠心後、細胞ペレットを500μLのPBS-5% FCS-2mM EDTAに懸濁し、さらに染色を行った。
免疫表現型解析とフローサイトメトリー解析
末梢リンパ節、腸間膜リンパ節、脾臓および腸組織(固有層および上皮内リンパ球)から単離した細胞を、Live/dead fixable viability dye(Ebioscience/Thermofisher)と適切なコンジュゲート抗TCRβで染色した、 CD45、CD19、CD4、CD8、CD11b、Ly6G、Ly6C、FcεRIa、F4/80、CD117(eBioscience/ThermofisherまたはBecton Dickinson)を1: 200希釈(mAb)、総容量50-100μlで、前述のように行った。細胞は、2%FBSを含むPBS中、暗所、4℃で20分間インキュベートした後、フローサイトメトリーで評価する前に、同じ培地で300g、5分間で1回洗浄した。ILC染色では、細胞を系統カクテルで染色し、系統陰性のCD45+細胞はCD127の発現を評価し、Gata-3(クローンL50-823)、RoRγtおよびTbx21の細胞内発現は固定/透過化後に行った(eBioscience/Thermofisher)。染色された細胞はフローサイトメトリー(LSR Fortessa, Becton Dickinson, San Jose, CA)によって評価され、各染色について最低10,000イベントが記録された。データ解析はFlowJoソフトウェア(Tree Star, Ashland, OR)を用いて行った。
ACh定量
選別した既知数の房細胞(純粋なメタノールで回収)と腸管洗浄液サンプル(0.2mL、すぐに0.8mLの純粋なメタノールと混合)を-80℃で48時間保存し、タンパク質を沈殿させた。次に、2-morpholinoethansulfonic acid (Cat. # 341-01622; Dojindo, Tokyo, Japan)を0.5μMの最終濃度(FC)で品質管理(QC)標準として添加し、サンプルにギ酸を1%FCまで添加した。サンプルを1分間激しくボルテックスした後、20.000xgで10分間遠心し、タンパク質を沈殿させた。0.9 mL の上清を、Vacuum Manifold (Agilent, Cat.# A796) に組み立てた Captiva EMR プレート (Agilent, Santa Clara, USA, Cat.# 5190-1001) に、Deep Well コレクションプレート (Agilent, Cat.# A696001000) とともにセットした。フロースルー画分は、まずSpeedvac concentrator(Thermo Scientific、Cat.# SPD2030)を用いて乾燥させ、その後200μLの水(Biosolve BV、Cat.# 0023214102BS)に懸濁した。検量線は、定義された量のAChをマトリックス(KOマウスの腸管洗浄により生成)で希釈することにより確立した。各サンプルからのタンパク質量は、BCAキット(Thermo Scientific)を用いて、製造者の推奨に従って測定した。簡単に説明すると、タンパク質ペレットを0.3mLの水に溶かした2%ドデシル硫酸ナトリウム溶液(Sigma Aldrich, Cat. その後、各サンプルのタンパク質含量を推定するために、2μLのサンプルを使用した。
再懸濁した Captiva フロースルー画分 1μL または標準物質 1μL を、UHPLC (Agilent, 1290 Infinity II Biocompatible) とトリプル四重極 MS (Agilent, 6495C) で構成される LC-MS システムに注入しました。サンプルはMRMs取得モードで分析された。ACh の定量には、147.1 → 43.0, 147.1 → 88.1, 147.1 → 87.1(ポジティブモード)のトランジションが用いられた。2-モルフォリノエタンスルホン酸のトランジションは以下の通りであった: 196.2 → 100.0(陽性)、194 → 80.15(陰性)。保持時間は次のように設定した: 2-モルフォリノエタンスルホン酸は2.04分、AChは8分とした。分析カラムはUPLC DiscoveryカラムHS F5-3 (Cat. # 567503-U; Sigma Aldrich)を用いた。移動相の組成は以下の通り: A:99.9%水(Biosolve BV、Cat. # 0023214102BS)、0.1%ギ酸(Sigma Aldrich、Cat. # 33015);B:99.9%アセトニトリル(Biosolve BV、Cat. # 001204102BS)、0.1%ギ酸。グラジエントは0分(100%A)、2分(100%A)、5分(75%A)、11分(65%A)、15分(5%A)、25分(5%A)、25.10分(100%A)、35分(100%A)。流速は0.25 mL/分、カラム温度は40℃に設定した。以下のソースパラメーターを使用した: ガス温度: ガス温度:150℃;ガスフロー:11 L/分;ネブライザー:40 psi;シースガス温度:0.25 mL/分;温度カラム:40℃: 40psi;シースガス温度 ガス温度:150℃;ガスフロー:11L/min;ネブライザー:40psi;シースガス温度:400℃;シースガスフロー:12L/min;キャピラリー電圧(ネガティブモード):4000V: 4000 V;キャピラリー電圧(ポジティブモード):4000 V 4000 V;ノズル電圧:500 V;IFunnel高圧RF Pos: 100 V ネガ: 50 V; IFunnel Low Pressure RF Pos: 100 V Neg: 50 V。
分析後、Agilent Masshunter Quantitative Analysis ソフトウェア(バージョン 10.1.733.0)を用いてピーク積分を行いました。AChの絶対定量は、各サンプルのピーク面積をACh検量線に当てはめることで、先に示したように算出した46。
RNA抽出とPCR
腸組織からの全RNAは、TRIzol(Life Technologies)を用いて単離した。RNeasy MicroおよびMini Kitカラム(Qiagen)も、細胞選別実験からのRNA精製に使用した。選別された細胞RNAは、Arcturus RiboAmp Plus kit(ThermoFischer Scientific, KIT0501)を用いて、製造者の指示に従って増幅した。逆転写は、Transcriptor First Strand cDNA synthesis KIT (Roche)を用いて、1μgの精製RNAを用い、メーカーの指示に従って行った。リアルタイム定量は、GapdhとHprtの平均Ctを内部ローディング対照として、5ngのRT産物についてLightCycler480 SYBR Green I Master(Roche)を用いて3連で行い、相対発現の算出にはΔΔCt法を用いた。
パラフィン包埋組織での蛍光免疫組織化学またはin situハイブリダイゼーション
パラフィン包埋組織の薄切片に対する組織解剖、固定、および免疫組織化学は、基本的に以前に記載されたとおりに行った47。エピトープ回収は、クエン酸ナトリウム(pH6.4)中10mMで20分間煮沸することにより達成した。この研究で使用した一次抗体は、4℃でONインキュベートし、主要リソース表に記載した。その後、TBS-Triton X-100 0.1%(シグマアルドリッチ)中の蛍光色素標識二次抗体(Jackson ImmunoResearch Laboratories, Inc.)および2μg/mL(シグマアルドリッチ)のDAPI、またはDAB(シグマ)で明らかにしたHRP標識二次抗体でインキュベートする前に、スライドを0.1% PBS-Tween(シグマアルドリッチ)で2回洗浄した。スライドはFluoroMount(Sigma)またはPertex(Histolab)でマウントし、それぞれ蛍光または可視イメージングを行った。mRNA in situ ハイブリダイゼーションでは、Chat mRNA(Cat No.408731、ACDBio)、SucnR1(Cat No.437721)、Ltc4s(Cat No.1046751)を標的とするプローブで組織をハイブリダイゼーションした。Dclk1の免疫蛍光検出は、上記の方法に従って行った。
顕微鏡とイメージング
蛍光写真は、カメラ(AxioCam MRm; Carl Zeiss, Inc.)、EC Plan Neofluar(5X NA 0.16; 10X NA 0.3、20X NA 0.5)を搭載したAxioImager Z1顕微鏡(Carl Zeiss, Inc.)で室温で取得した。 3; 20X NA 0.5; 100X NA 1.3)およびPlan Apochromat (40X NA 0.95; 63X NA 1.4)レンズ、H1透過グリッドを装備したアポトームスライダーシステム(Carl Zeiss, Inc.)、およびZenソフトウェア(Carl Zeiss, Inc.)を搭載。写真の後処理(レベル補正)、注釈、パネル構成は、Photoshop(Adobe)またはZen(Carl Zeiss)ソフトウェアを用いて行った。明視野免疫組織化学写真は、Plan Fluor (10X NA 0.3; 20X NA 0.5; 40X NA 0.75; and 60X NA 0.5-1.25)レンズ(Nikon)とデジタルカメラ(Q-Imaging Retiga 2000R with a Q-Imaging RGB Slider)を装着したEclipse 80i顕微鏡(Nikon)を用い、Q-Capture Proソフトウェア(Nikon)を用いて室温で撮影した。染色スライドは、Nanozoomer装置(浜松ホトニクス製)でも撮像し、NdpViewソフトウェア(浜松ホトニクス製)で可視化し、注釈を付けた。
定量化と統計解析
記述統計解析にはPrismソフトウェアを使用した。正規分布検定後、データセットは両側Student T検定(正規分布、一対比較)またはノンパラメトリック両側Mann-Whitney U検定(非正規分布、一対比較)で解析した。多重比較のために、データセットは一元配置ANOVA分析(正規分布、Benjamini-Yekutieliポストホック補正あり)またはKruskal-Wallis分析(非正規分布、Benjamini-Yekutieliポストホック補正あり)で進められた。いくつかの分析では、複数のMann-Whitney分析を行った。正規分布に応じて、データセットは各グラフにおいて、平均値±SDに対応する個々の値および棒グラフ(正規分布)、または中央値±四分位数に対応する個々の値および棒グラフ(非正規分布)のいずれかで表される。統計解析の詳細は各図の凡例に記載されている。
謝辞
M.Selkirk教授には科学的な議論について、E.Valjent博士には技術的なインプットと試薬について、Elisa Evainにはイムノフェノタイピング実験について、RAM-iExploreおよびRAM-PCEA施設にはマウスコロニーの維持について、Montpellier RIO ImagingおよびArpege施設には感謝する。この研究は、P.J.、C.B.、E.D.、T.M.、R.M.に対するWellcome Trust Collaborative Award(ref.211814)の支援を受けている、 およびR.M.、Agence Nationale de la Recherche (ANR-17-CE15-0016-01 and ANR-21-CE15-0017-01 to P.J.)、Institut National Du Cancer (INCA_2018-158 to P.J.)-P.J.チーム。チームは "Equipe Labellisée Ligue contre le Cancer "である。M.N.はLabex EpiGenMed("Investissements d'avenir "プログラムANR-10-LABX-12-01)およびWellcome Trust Collaborative Award(ref.211814)の支援を受けた。CPER助成金(IBDLR)を通じてPLATON施設を支援してくれたフランス高等教育・研究省、および技術的な議論をしてくれたOriane Scholler(PLATON)に謝意を表する。
著者の貢献
構想、P.J.、F.G.、R.M.M.、方法論、M.D.、F.H.、C.J.、A.T.、S.T.、調査、M.D.、F.G.、F.H.、I.G.、C.J.、J.B、 A.L.、N.C.、S.H.、V.D.、V.S.Z.、M.C.P.、E.T.、A.G.、C.C.、原案執筆:P.J.、F.G.、査読・編集:P.J、 F.G.、R.M.M.、C.B.、E.D.、T.N.M.、V.D.、V.S.Z.、A.T.、S.B.、資金獲得、R.M.M.、P.J、 C.B.、E.D.、T.N.M.、リソース、S.B.、Z.H.、J.P.、L.F.、C.C.、監督、P.J.、F.G.、R.M.M.。
利益申告
著者らは、競合する利益はないと宣言している。
補足情報
pdfをダウンロード (11.92 MB)
pdfファイルのヘルプ
ドキュメントS1. 図S1-S7
参考文献
ゲルベ F.
シドー E.
スマイス D.J.
オオモト M.
松本 I.
ダーダルホン V.
セス P.
ガルニエ L.
プゾル M.
ブルーリンB.
et al.
腸管上皮房細胞は蠕虫寄生虫に対する2型粘膜免疫を開始する。
Nature. 2016; 529: 226-230
https://doi.org/10.1038/nature16527
論文で見る
スコープス (624)
PubMed
クロス
グーグル奨学生
ハウィット M.R.
ラヴォアS.
ミショー M.
ブルーム A.M.
トラン S.V.
ワインストックJ.V.
ガリーニ C.A.
レディング K.
マーゴルスキー R.F.
オズボーンL.C.
他
味覚化学感覚細胞であるタフト細胞は、腸内寄生虫2型免疫を制御している。
Science. 2016; 351: 1329-1333
https://doi.org/10.1126/science.aaf1648
論文で見る
スコープス (620)
PubMed
クロス
グーグル奨学生
フォンモルトケJ.
Ji M.
Liang H.-E.
Locksley R.M.
タフト細胞由来IL-25は、腸管ILC2-上皮応答回路を制御する。
Nature. 2016; 529: 221-225
https://doi.org/10.1038/nature16161
論文で見る
スコープス (832)
PubMed
クロス
グーグル奨学生
アルティス D.
ワン M.L.
キールボー S.A.
He W.
ブレネス M.
スウェイン G.P.
ナイト P.A.
ドナルドソン D.D.
ラザール M.A.
ミラーH.R.P.
ほか
RELMbeta/FIZZ2は消化管における杯細胞特異的免疫エフェクター分子である。
Proc. Natl. Sci. USA. 2004; 101: 13596-13600
https://doi.org/10.1073/pnas.0404034101
論文で見る
スコープス (275)
PubMed
クロス
グーグル奨学生
ハーバート D.R.
ヤン J.Q.
ホーガン S.P.
グロシュヴィッツ K.
Khodoun M.
ムニッツ A.
オレコフ T.
パーキンス C.
ワン Q.
ブロムバッハーF.
他。
RELM-βの腸管上皮細胞分泌は、消化管ミミズの感染を防御する。
J. Exp. Med. 2009; 206: 2947-2957
https://doi.org/10.1084/jem.20091268
論文で見る
スコープス(211)
パブコメ
クロス
グーグル奨学生
チェン・G.
Wang S.H.
Jang J.C.
オデガード J.I.
ネール M.G.
RELMαとRELMβの単一遺伝子欠損マウスと二重遺伝子欠損マウスの比較から、RELMαの発現が鉤虫感染における炎症と虫の排出を規定することが明らかになった。
Infect. Immun. 2016; 84: 1100-1111
https://doi.org/10.1128/IAI.01479-15
論文で見る
スコープス (29)
PubMed
クロス
グーグル奨学生
モロフスキーA.B.
ロックスリー R.M.
自然免疫と適応2型免疫の内と外。
Immunity. 2023; 56: 704-722
https://doi.org/10.1016/j.immuni.2023.03.014
論文で見る
スコパス (14)
パブコメ
概要
全文
全文PDF
グーグル奨学生
ファロン P.G.
バランタイン S.J.
マンガン N.E.
バーロウ J.L.
ダスバルマ A.
ヒューエット D.R.
マキルゴーム A.
ジョリン H.E.
マッケンジー A.N.J.
蠕虫駆除開始時にIL-4、IL-5、IL-13を供給するインターロイキン(IL)-25依存性細胞集団の同定。
J. Exp. Med. 2006; 203: 1105-1116
https://doi.org/10.1084/jem.20051615
論文で見る
スコープス (617)
PubMed
クロス
グーグル奨学生
ウェスラーI.
カークパトリックC.J.
コリン作動性シグナルは免疫機能を制御し、恒常性を促進する。
Int. Immunopharmacol. 2020; 83106345
https://doi.org/10.1016/j.intimp.2020.106345
論文で見る
スコープス (9)
PubMed
クロス
グーグル奨学生
クラステヴァ G.
カニングB.J.
ハルトマンP.
ヴェレスT.Z.
パパダキス T.
ミュールフェルド C.
シュリーカー K.
タリーニ Y.N.
ブラウン A.
ハックシュタインH.
他
気管のコリン作動性化学感覚細胞は呼吸を制御する。
Proc. Natl. Sci. USA. 2011; 108: 9478-9483
https://doi.org/10.1073/pnas.1019418108
論文で見る
スコープス (228)
PubMed
クロス
グーグル奨学生
デックマンK.
フィリプスキーK.
クラステヴァ・クリストG.
フロニウス M.
アルタウス M.
ラフィク A.
パパダキス T.
レンノ L.
ジュラストウ I.
ヴェッセルスL.
ら。
苦味は、ポリモーダル尿道化学感覚細胞からのアセチルコリン放出と膀胱反射を誘発する。
Proc. Natl. Acad. Sci. USA. 2014; 111: 8287-8292
https://doi.org/10.1073/pnas.1402436111
論文で見る
スコープス (128)
PubMed
クロス
グーグル奨学生
シュッツB.
ジュラストウI.
バーダーS.
リンガー C.
フォンエンゲルハルトJ.
チュバノフ V.
グーデルマン T.
ディーナー M.
クマー W.
クラステヴァ・クリスト G.
ヴァイエ E.
マウスの消化管および胆道におけるコリン作動性ブラシ細胞の化学コード化と化学感覚特性。
Front. Physiol.
https://doi.org/10.3389/fphys.2015.00087
論文で見る
スコープス (96)
PubMed
クロス
グーグル奨学生
パンネック A.R.
ラフィクA.
シュッツB.
Soultanova A.
デックマン K.
チュバノフ V.
グデルマン T.
ヴァイエ E.
クラステヴァ・クリスト G.
グラウ V.
他。
マウス胸腺髄質における化学感覚特性を持つコリン作動性上皮細胞。
Cell Tissue Res: 737-748
https://doi.org/10.1007/s00441-014-2002-x
論文で見る
スコパス (51)
PubMed
クロス
グーグル奨学生
ペルニス A.
リュー S.
ブーネンB.
Keshavarz M.
ルパート A.-L.
ティム T.
プファイル U.
ソウルタノワ A.
クスマクシ S.
デルヴェンタールL.
他
化学感覚細胞由来アセチルコリンは、ウイルス性関連ホルミルペプチドに応答して気管粘膜繊毛クリアランスを促進する。
Immunity. 2020; 52: 683-699.e11
https://doi.org/10.1016/j.immuni.2020.03.005
論文で見る
スコープス (56)
パブコメ
概要
全文
全文PDF
グーグル奨学生
サンダース C.J.
クリステンセン M.
フィンガーT.E.
ティッツァーノM.
コリン作動性神経伝達が孤発性化学感覚細胞と鼻炎を結びつけている。
Proc. Natl. Sci. USA. 2014; 111: 6075-6080
https://doi.org/10.1073/pnas.1402251111
論文で見る
スコープス (159)
PubMed
クロス
グーグル奨学生
クラステヴァ G.
カニングB.J.
パパダキスT.
クマーW.
気管のコリン作動性ブラシ細胞は、クオラムセンシング分子に対する呼吸応答を媒介する。
生命科学 2012; 91: 992-996
https://doi.org/10.1016/j.lfs.2012.06.014
論文で見る
スコープス (72)
PubMed
クロス
グーグル奨学生
ホレンホルスト M.I.
ジュラストウI.
ナンディガマR.
アッペンツェラー S.
Li L.
フォーゲル J.
ヴィーダーホルド S.
アルタウス M.
エンプティング M.
アルトミュラーJ.
他
気管ブラシ細胞は、苦味物質に反応してアセチルコリンを放出し、パラクリンおよびオートクリンシグナル伝達を行う。
faseb j. 2020; 34: 316-332
https://doi.org/10.1096/fj.201901314RR
論文で見る
スコープス (39)
PubMed
クロス
グーグル奨学生
マーティン R.J.
ロバートソン A.P.
レバミソールとピランテルの作用機序、駆虫薬耐性、E153とQ57。
Parasitology. 2007; 134: 1093-1104
https://doi.org/10.1017/S0031182007000029
論文で見る
スコパス (68)
PubMed
クロス
グーグル奨学生
セルカーク M.E.
ラザリO.
フセイン A.S.
マシューズ J.B.
線虫アセチルコリンエステラーゼは複数の遺伝子にコードされ、重複しない機能を果たす。
Chem. Biol. Interact. 2005; 157-158: 263-268
https://doi.org/10.1016/j.cbi.2005.10.039
論文で見る
スコープス (22)
PubMed
クロス
グーグル奨学生
レイノルズL.A.
フィルベイK.J.
Maizels R.M.
モデル腸管蠕虫寄生虫Heligmosomoides polygyrusに対する免疫。
Semin. Immunopathol. 2012; 34: 829-846
https://doi.org/10.1007/s00281-012-0347-3
論文で見る
スコープス (156)
PubMed
クロス
グーグル奨学生
Drurey C.
リンドホルムH.T.
コークリーG.
ポベダ M.C.
Löser S.
ドゥーラン R.
ゲルベ F.
ジェイ P.
ハリス N.
ウードホフ M.J.
マイゼルスR.M.
腸管上皮房細胞誘導はネズミの蠕虫とその分泌産物によって阻害される。
J. Exp. Med. 2022; 219e20211140
https://doi.org/10.1084/jem.20211140
論文で見る
スコープス (37)
PubMed
クロス
グーグル奨学生
ルコント M.-J.
ベルトルス C.
サンタマリアJ.
ボーシェ A.-L.
ヘルバン M.
サウリーニ F.
三澤秀雄
メゾノベ T.
プラダ P.-F.
ノステン・ベルトラン M.
他
運動ニューロンのサブセットにおけるアセチルコリン合成の選択的阻害:遅発性運動ニューロン疾患の新しいモデル。
Neurobiol. Dis. 2014; 65: 102-111
https://doi.org/10.1016/j.nbd.2014.01.014
論文で見る
スコープス (7)
PubMed
クロス
グーグル奨学生
エル・マルジュー F.
ヤンセン K.-P.
チャン B.H.J.
リー M.
ヒンディー V.
チャン L.
ルーバード D.
シャンボン P.
メッツガー D.
ロビーン S.
腸上皮における組織特異的かつ誘導可能なCre媒介組換え。
genesis. 2004; 39: 186-193
https://doi.org/10.1002/gene.20042
論文で見る
日本
PubMed
クロス
グーグル奨学生
ナジソンバティ M.S.
マクギンティJ.W.
ライオンズ-コーエンM.R.
ジャッフェ J.B.
ディペソL.
シュナイダー C.
ミラー C.N.
ポラック・J.L.
ナガナ・ゴウダ G.A.
フォンタナ M.F.
他
腸房細胞によるコハク酸の検出は、2型自然免疫回路を誘発する。
Immunity. 2018; 49: 33-41.e7
https://doi.org/10.1016/j.immuni.2018.06.016
論文で見る
スコパス (326)
PubMed
要旨
全文
全文PDF
グーグル奨学生
シュナイダー C.
オリアリーC.E.
フォン・モルトケJ.
Liang H.-E.
アンQ.Y.
ターンボー P.J.
ラダクリシュナン S.
ペリゾン M.
マー A.
ロックスリー R.M.
メタボライトをトリガーとするタフト細胞-ILC2回路が小腸のリモデリングを促進する。
Cell. 2018; 174: 271-284.e14
https://doi.org/10.1016/j.cell.2018.05.014
記事で見る
スコープス (282)
パブコメ
要旨
全文
全文PDF
グーグル奨学生
インクラン-リコJ.M.
シラクサ M.C.
ファーストレスポンダー:蠕虫に対する自然免疫。
Trends Parasitol. 2018; 34: 861-880
https://doi.org/10.1016/j.pt.2018.08.007
論文で見る
スコパス (58)
PubMed
概要
全文
全文PDF
グーグル奨学生
セルカーク M.E.
ラザリO.
マシューズ J.B.
線虫アセチルコリンエステラーゼの機能ゲノミクス。
Parasitology. 2005; 131: s3-s18
https://doi.org/10.1017/S0031182005008206
論文で見る
(41件)
PubMed
クロス
グーグル奨学生
ビリップ T.E.
ファングC.
ウェベックL.M.
サージェントD.B.
ゴロゴロスキーM.B.
マクダニエル M.M.
カサル D.N.
マクギンティJ.W.
バロー K.A.
リッチL.M.
他
タフト細胞由来のアセチルコリンが上皮液分泌を制御する。
bioRxiv. 2023; (Preprint at)
https://doi.org/10.1101/2023.03.17.533208
論文で見る
哺乳類
PubMed
クロス
グーグル奨学生
リュー S.
Lu S.
Xu R.
Atzberger A.
ギュンター S.
ヴェッツシュレック N.
オッフェルマンズ S.
苦味受容体クラスターTas2r143/Tas2r135/Tas2r126のメンバーは、マウスの気道および他の非味覚組織の上皮に発現している。
Front. Physiol.
https://doi.org/10.3389/fphys.2017.00849
論文で見る
スコパス (36)
クロス
グーグル奨学生
バニー I.A.
ドン M.-Q.
コエル M.R.
線虫の産卵行動におけるアセチルコリン阻害の遺伝学的および細胞学的基盤。
J. Neurosci. 2003; 23: 8060-8069
https://doi.org/10.1523/JNEUROSCI.23-22-08060.2003
論文で見る
パブコメ
クロスフィルム
グーグル奨学生
You H.
リウ C.
Du X.
マクマナスD.P.
シストソームおよびその他の寄生蠕虫におけるアセチルコリンエステラーゼとニコチン性アセチルコリン受容体。
Molecules. 2017; 22: 1550
https://doi.org/10.3390/molecules22091550
記事で見る
スコパス (10)
PubMed
クロス
グーグル奨学生
ロサス-バリナ M.
トレーシーK.J.
炎症のコリン作動性制御。
J. インターン。Med. 2009; 265: 663-679
https://doi.org/10.1111/j.1365-2796.2009.02098.x
記事で見る
スコープス (453)
PubMed
クロス
グーグル奨学生
ダービー M.
シュノラーC.
ビラ A.
カリー F.J.
ボバットS.
ローガン E.
キルシュタイン F.
ウェス J.
カニンガム A.F.
ブロムバッハー F.
et al.
M3ムスカリン受容体は蠕虫および細菌感染に対する最適な適応免疫に必要である。
PLoS Pathog. 2015; 11e1004636
https://doi.org/10.1371/journal.ppat.1004636
論文で見る
スコープス (38)
PubMed
クロス
グーグル奨学生
ヴォーR.
シュノラーC.
Berkachy R.
ロバーツ L.B.
ハーゲンJ.
グナリス K.
セルカーク M.E.
線虫分泌アセチルコリンエステラーゼによる免疫応答の調節がTrypanosoma musculiにおける異種発現によって明らかになった。
PLoS Pathog. 2016; 12e1005998
https://doi.org/10.1371/journal.ppat.1005998
論文で見る
スコパス (23)
PubMed
クロス
グーグル奨学生
チュー C.
パークハースト C.N.
Zhang W.
Zhou L.
Yano H.
アリフザマン M.
アーティスD.
ChAT-アセチルコリン経路は、2群自然リンパ球応答と抗蠕虫免疫を促進する。
Sci. Immunol. 2021; 6eabe3218
https://doi.org/10.1126/sciimmunol.abe3218
論文で見る
スコパス (60)
クロスリファレンス
グーグル奨学生
ロバーツ L.B.
シュノラーC.
バーカシーR.
ダービーM.
ピレイ J.
ウードホフ M.J.
パルマー N.
マコウィアック C.
セッダ D.
ケスニアーV.
他
2群自然リンパ球によるアセチルコリン産生は蠕虫に対する粘膜免疫を促進する。
Sci. Immunol. 2021; 6eabd0359
https://doi.org/10.1126/sciimmunol.abd0359
論文で見る
スコパス (42)
クロスリファレンス
グーグル奨学生
ヘップワース M.R.
ダニウォヴィッチ・ルーベールE.
ラウシュ S.
メッツ M.
クロッツ C.
マウラー M.
ハルトマン S.
マスト細胞は、組織由来サイトカインの制御を介して蠕虫に対する2型免疫を制御する。
Proc. Natl. Acad. Sci. 2012; 109: 6644-6649
https://doi.org/10.1073/pnas.1112268109
論文で見る
スコープス (133)
パブコメ
クロス
グーグル奨学生
下川 慈
金谷武志
蜂須賀雅之
石渡和彦
久枝裕子
倉島康弘
清野秀之
吉本貴志
海翔T.
大野裕之
肥満細胞は2群自然リンパ球の誘導と蠕虫感染の除去に重要である。
Immunity. 2017; 46: 863-874.e4
https://doi.org/10.1016/j.immuni.2017.04.017
論文で見る
スコープス (138)
PubMed
概要
全文
全文PDF
グーグル奨学生
アリザデ H.
マレル K.D.
肥満細胞欠損w/wvマウスにおけるTrichinella spiralis感染に対する腸肥満細胞反応。
J. Parasitol. 1984; 70: 767-773
論文で見る
PubMed
クロスフィルム
グーグル奨学生
ゴンサレス M.I.
ロペスF.
マッケイ D.M.
レイエス J.L.
マウスの肥満細胞欠損によるラット条虫Hymenolepis diminutaのバイオマス過剰増殖と排出遅延。
Biosci. Rep. 2018; 38BSR20180687
https://doi.org/10.1042/BSR20180687
論文で見る
スコパス (8)
Crossref
グーグル奨学生
ドナルドソン L.E.
シュミットE.
ハントリーJ.F.
ニューランズ G.F.
グレンシス R.K.
腸管蠕虫に対する宿主防御免疫における幹細胞因子とc-kitの重要な役割。
Int. Immunol. 1996; 8: 559-567
https://doi.org/10.1093/intimm/8.4.559
論文で見る
スコープス (101)
PubMed
クロス
グーグル奨学生
ツァイ M.
ヴァレント P.
ガリ S.J.
肥満細胞系譜のマスターレギュレーターとしてのKIT。
J. Allergy Clin. Immunol. 2022; 149: 1845-1854
https://doi.org/10.1016/j.jaci.2022.04.012
論文で見る
スコープス (31)
パブコメ
概要
全文
全文PDF
グーグル奨学生
シュミット M.
シェヴェ M.
サチェッティA.
フェイテルD.
ファン・デ・ギールW.S.
ティーウッセン M.
スレデンス H.F.
ヨーステン R.
ファン・ロイエン M.E.
ファン・デ・ヴェルケンH.J.G.
他
パネス細胞は炎症に応答し、SCF/c-Kitシグナルを通じて幹様機能を獲得することで組織再生に寄与する。
Cell Rep. 2018; 24: 2312-2328.e7
https://doi.org/10.1016/j.celrep.2018.07.085
論文で見る
スコープス (148)
PubMed
要旨
全文
全文PDF
グーグル奨学生
ローテンバーグ M.E.
ヌッセ Y.
カリスキーT.
リーJ.J.
ダレルバ P.
シェーレン F.
ロボ N.
クルカルニ S.
シム S.
Qian D.
et al.
マウスのLgr5+幹細胞をサポートするCkit+大腸クリプト基底分泌細胞の同定。
Gastroenterology. 2012; 142: 1195-1205.e6
https://doi.org/10.1053/j.gastro.2012.02.006
論文で見る
スコープス (210)
パブコメ
概要
全文
全文PDF
グーグル奨学生
ハーバーA.L.
ビトン M.
ロゲル N.
ハーブスト R.H.
シェカールK.
スミリー・C
バージン G.
デロリー T.M.
ハウィット M.R.
カッツY.
他。
小腸上皮の単一細胞調査。
Nature. 2017; 551: 333-339
https://doi.org/10.1038/nature24489
論文で見る
スコープス (954)
PubMed
クロス
グーグル奨学生
トゥルトイ E.
ジュディ J.
ヘンリー S.
ダディ I.
ヴァレットG.
エンジャルバル C.
Turtoi A.
ターゲットメタボロミクスとLC-MSを用いた生体試料中の極性一次代謝物の解析。
STAR Protoc. 2023; 4102400
https://doi.org/10.1016/j.xpro.2023.102400
論文で見る
スコープス (2)
クロスリファレンス
グーグル奨学生
ゲルベ F.
ファン・エスJ.H.
マクリーニL.
ブルーリンB.
メリッツァーG.
ロビネ S.
ロマニョーロ B.
シュロイヤー N.F.
ブルゴー J.-F.
ピグノーデルC.
他
ATOH1とNeurog3の必要性が異なることから、タフト細胞は腸上皮における新しい分泌細胞タイプであることが明らかになった。
J. Cell Biol.
https://doi.org/10.1083/jcb.201010127
論文で見る
スコパス(304)
PubMed
クロス
グーグル奨学生
論文情報
出版履歴
出版 2024年6月11日
受理 受理:2024年4月17日
改訂版受理 2023年11月26日
受理:2023年11月26日 受理日:2023年6月18日
識別
DOI: https://doi.org/10.1016/j.immuni.2024.04.018
著作権
© 2024 The Authors. 発行:エルゼビア社
ユーザーライセンス
クリエイティブ・コモンズ 表示 (CC BY 4.0)|情報アイコンの再利用方法
サイエンスダイレクト
ScienceDirectでこの論文にアクセスする
図
図サムネイルfx1
グラフィカルアブストラクト
サムネイルgr1
図1Chat遺伝子の発現は、ナイーブマウスおよびH. polygyrus感染時の腸管上皮の房細胞に限定される。
図サムネイルgr2
図2Villin-CreERT2遺伝子導入による腸管上皮細胞限定組換え。
図サムネイルgr3
図3Tuft細胞Chatの欠損は虫の排出を遅らせる
図サムネイルgr4
図4Chat遺伝子欠損は2型免疫応答の確立を阻害しない
図4Chat遺伝子欠損は2型免疫応答の確立を阻害しない
図5Tuft細胞に制限されたChat遺伝子欠損はH. polygyrus感染後の腸管肥満細胞症の確立を阻害する
サムネイルgr6
図6Tuft細胞由来のAChは宿主腸管内腔に放出され、AChは直接虫の生理機能を障害する。
図サムネイルgr7
図7蠕虫寄生虫感染時の房細胞エフェクター機能を示す模式図
関連記事
タフト細胞由来のアセチルコリンは上皮の塩化物分泌と腸管蠕虫のクリアランスを促進する
Billipp et al.
免疫2024年5月13日
イン・ブリーフ全文PDF
関連記事
広告
研究ジャーナル
細胞
癌細胞
細胞化学生物学
細胞ゲノム
細胞宿主と微生物
細胞代謝
細胞レポート
セルレポーツ医学
セルレポートメソッド
セルレポート 物理科学
細胞幹細胞
細胞システム
化学
化学触媒
カレントバイオロジー
発生細胞
ヘリオン
免疫
アイサイエンス
ジュール
物質
医学
分子細胞
ニューロン
一つの地球
パターン
STARプロトコル
構造
トレンドレビュージャーナル
生化学
バイオテクノロジー
癌
細胞生物学
化学
認知科学
生態学・進化学
内分泌学・代謝学
遺伝学
免疫学
微生物学
分子医学
神経科学
寄生虫学
薬理学
植物科学
パートナージャーナル
AJHG
生物物理ジャーナル
生物物理学レポート
EBioMedicine
HGGアドバンス
分子植物
分子療法ファミリー
植物通信
幹細胞レポート
イノベーション
著者
論文投稿
複数ジャーナルへの投稿
STARメソッド
プレビュー - プレプリント
査読者
査読者向け情報
ニュース&イベント
ニュースルーム
細胞シンポジウム
コンソーシアムハブ
ウェビナー
ラボリンク
マルチメディア
セルプレスポッドキャスト
セルプレスビデオ
カラーリングとコミック
フィギュア360
セル画ショー
研究篇
セルプレスについて
セルプレスについて
オープンアクセス
COVIDハブ
持続可能性
インクルージョンと多様性
コンタクト
お問い合わせ
ヘルプ&サポート
採用情報
セルプレス採用情報
サイエンティフィックジョブボード
アクセス
登録する
請求
今すぐ読む
司書に推薦する
出版アラート
コレクション
ベスト・オブ・セルプレス
セルプレスレビュー
セルプレスセレクション
Nucleusコレクション
スナップショット・アーカイブ
インフォメーション
広告主の皆様へ
リクルーターの方へ
図書館員の方へ
プライバシーポリシー
ご利用条件
アクセシビリティ
本サイトのコンテンツは、あらゆる分野の医療従事者および研究者を対象としています。
当サイトでは、サービスの提供・向上やコンテンツのカスタマイズのためにクッキーを使用しています。クッキーの設定を更新するには、このサイトのクッキー設定をご覧ください。
このサイトのすべてのコンテンツ Copyright © 2024 Elsevier Inc.、そのライセンサー、および寄稿者。
テキストマイニング、データマイニング、AIトレーニング、および同様の技術に関するものも含め、すべての権利はエルゼビア社に帰属します。
すべてのオープンアクセスコンテンツには、クリエイティブ・コモンズのライセンス条件が適用されます。
プライバシーポリシー 利用規約 アクセシビリティ ヘルプ&サポート お問い合わせ
RELXSkip to Main ContentSkip to Main Menu
Main menu
ARTICLE| VOLUME 57, ISSUE 6, P1260-1273.E7, JUNE 11, 2024
Download Full Issue
Tuft cell acetylcholine is released into the gut lumen to promote anti-helminth immunity
Open AccessPublished:May 13, 2024DOI:https://doi.org/10.1016/j.immuni.2024.04.018
Highlights
•
Tuft cells play both sentinel and effector roles in type 2 immune responses
•
Tuft cell acetylcholine production increases during helminth infections
•
Tuft cells release acetylcholine into the gut lumen during type 2 immune responses
•
Acetylcloline inhibits helminth fecundity through worm muscarinic receptors
Summary
Upon parasitic helminth infection, activated intestinal tuft cells secrete interleukin-25 (IL-25), which initiates a type 2 immune response during which lamina propria type 2 innate lymphoid cells (ILC2s) produce IL-13. This causes epithelial remodeling, including tuft cell hyperplasia, the function of which is unknown. We identified a cholinergic effector function of tuft cells, which are the only epithelial cells that expressed choline acetyltransferase (ChAT). During parasite infection, mice with epithelial-specific deletion of ChAT had increased worm burden, fitness, and fecal egg counts, even though type 2 immune responses were comparable. Mechanistically, IL-13-amplified tuft cells release acetylcholine (ACh) into the gut lumen. Finally, we demonstrated a direct effect of ACh on worms, which reduced their fecundity via helminth-expressed muscarinic ACh receptors. Thus, tuft cells are sentinels in naive mice, and their amplification upon helminth infection provides an additional type 2 immune response effector function.
Graphical abstract
Keywords
Introduction
Tuft cells are a cellular subset mostly found in digestive and respiratory epithelia and play critical roles in mucosal host defense. In the intestinal epithelium, tuft cells are primarily known for their critical sentinel function during parasite infections. The presence of helminth or protozoa in the gut triggers tuft cell secretion of the alarmin cytokine interleukin-25 (IL-25) that initiates a type 2 immune response.
1
,
2
,
3
Such a response is principally orchestrated by type 2 innate lymphoid cells (ILC2s) through the secretion of type 2 cytokines such as IL-4, IL-5, and IL-13. A critical aspect of IL-4 and IL-13 function is the profound remodeling they cause in the intestinal epithelium. This includes amplification of the mucus-producing goblet cells and the tuft cell lineages, as well as resistin-like beta (Retnlβ) ectopic expression by small intestinal goblet cells, in which it is usually absent.
4
While Retnlβ directly interferes with worm physiology,
4
,
5
,
6
increased mucus production and smooth muscle hypercontractility, also known as the “weep and sweep” response, facilitate worm expulsion.
7
In contrast, the physiological role of the dramatic increase in tuft cell numbers during type 2 immune responses is not yet understood. Increased IL-25 production following tuft cell lineage amplification is thought to lead to a more efficient type 2 immune response,
1
,
3
but alternative tuft cell functions also need to be considered. Firstly, we noted that this amplification occurred downstream of the action of type 2 cytokines on epithelial cells; secondly, we noted that worm expulsion was substantially more delayed by the absence of tuft cells
1
as compared to IL-25 deficiency alone. These points strongly suggest that, in addition to their alarm function,
8
tuft cells are required not only as initiators but rather as an integral effector component of the type 2 immune response.
The acetylcholine (ACh) neurotransmitter, biosynthesized by the choline acetyltransferase (ChAT) enzyme, regulates a variety of neuronal and non-neuronal physiological functions.
9
Studies with Chat reporter mice revealed the presence of cholinergic epithelial cells in various tissues including the trachea,
10
urethra,
11
gastrointestinal and biliary tracts,
12
and thymus.
13
These cells were identified as tuft (also called brush or solitary chemosensory) cells in the airway, where their actual ACh release was demonstrated.
14
In the nasal cavity, cholinergic tuft cells regulate breathing and inflammation in the presence of irritants
15
; in the trachea, they control breathing reflexes
16
and muco-ciliary clearance in response to bacterial quorum sensing molecules.
17
Additionally, urethral tuft cells control micturition reflexes through cholinergic signaling to viscerosensory neurons in response to exogenous bitter compounds.
11
In contrast, the function of intestinal ChAT-expressing cells remains unknown.
Interestingly, some commonly used drugs against intestinal helminths, such as levamisole and pyrantel, are cholinergic agonists. They target the worm ACh receptors (AChRs), causing spastic paralysis, which facilitates worm expulsion
18
and suggests potential direct effects of host ACh on parasites. In addition, available ACh for signaling results from the balance between its synthesis by the ChAT enzyme and its breakdown catalyzed by acetylcholinesterase (AChE) enzymes. It is striking that some parasitic nematodes that colonize mucosal surfaces encode additional AChE isoforms, which are produced in specific secretory glands and secreted into the worm environment
19
to most likely avoid detrimental exposure to host ACh.
Here, we investigated the specific role of tuft cell-derived ACh in the context of type 2 immune responses. We confirmed that tuft cells are the only intestinal epithelial cells expressing the key Chat gene for ACh biosynthesis, and they actually synthetize ACh. Worm clearance was delayed in mice with Chat-deficient intestinal epithelial cells in spite of the establishment of a strong type 2 immune response. Mechanistically, we demonstrated in vivo tuft cell ACh release into the gut lumen; ex vivo, we demonstrated a direct effect of ACh on worms, resulting in reduced fecundity via a muscarinic AChR-dependent pathway, as well as a transiently reduced mastocytosis in mice with Chat-deficient epithelial cells.
Results
The gene encoding the ChAT enzyme is specifically expressed by intestinal epithelial tuft cells
To confirm the specific potential of small intestinal tuft cells to biosynthesize the ACh neurotransmitter, we assessed the expression of the gene encoding the choline acetyltransferase (ChAT) enzyme that catalyzes biosynthesis of ACh in fluorescence-activated cell sorting (FACS)-enriched small intestinal EpCam+; Siglec-F+ tuft cells as compared to the EpCam+; Siglec-F− non-tuft epithelial cell fraction. Efficiency of the cell-sorting procedure was assessed by analyzing the expression of the tuft cell marker Trpm5 mRNA in the tuft and non-tuft cell fractions. The Trpm5 mRNA was detected in the tuft cell fraction, whereas it was below the threshold of detection in the fraction containing non-tuft cells (Figure 1A). Similarly, the Chat mRNA was detected in the tuft cell fraction but not in the fraction containing all non-tuft cell epithelial subsets (EpCam+; Siglec-F−) of the small intestinal epithelium, indicating specific expression of the Chat gene in tuft cells among intestinal epithelial cells in vivo (Figure 1B). Then, to assess whether Chat mRNA expression is a common property of small intestinal tuft cells or is limited to only a subset of these cells, in the context of an intact tissue, we coupled anti-doublecortin-like kinase 1 (Dclk1) immunofluorescence detection of the entire tuft cell population to in situ hybridization to visualize Chat-expressing cells in naive C57BL/6 mice. This revealed specificity of the Chat probe signal in the vast majority of Dclk1-expressing tuft cells (Figure 1C). We then asked whether Chat expression is also a common feature of the amplified tuft cell population in the context of a helminth parasite infection and an ongoing type 2 immune response.
1
,
3
Wild-type mice were thus infected by gavage with Heligmosomoides polygyrus infective L3 larvae, which, after reaching the small intestine, penetrate the submucosa. There, they undergo two developmental moults before emerging again around 10 days post-infection (dpi) as adult worms into the gut lumen, where they mate and produce eggs, which are passed out in the feces. The presence of H. polygyrus worms in the gut lumen was associated with a strongly polarized type 2 immune response,
20
which included amplification of tuft cells.
21
Chat mRNA expression was also restricted to tuft cells from H. polygyrus-infected mice (Figure 1D), indicating the absence of de novo Chat expression in non-tuft cells in the context of a type 2 immune response, and was detected in almost all tuft cells. Furthermore, a quantitative comparison revealed similar proportions of Chat+ Dclk1-expressing tuft cells in naive (574/614 examined cells) and infected (435/500 examined cells) mice (Figure 1E). Thus, Chat mRNA expression was present specifically in tuft cells in the mouse small intestinal epithelium and could be detected in almost all tuft cells regardless of their infection status.
Host defense against helminth parasites is impaired in ChAT-deficient mice
We then investigated the function of tuft cell-produced ACh in the context of an in vivo type 2 immune response. We generated an inducible deletion of the Chat gene specifically in the intestinal epithelium by crossing ChatLoxP/LoxP
22
and Villin-CreERT2 mice to express the Cre recombinase in intestinal epithelial cells in a tamoxifen-inducible manner.
23
To assess the efficiency of the Villin-CreERT2-mediated recombination at the Chat gene locus, we amplified by PCR the sequence of the LoxP-flanked exon 8 of the Chat gene in tamoxifen-treated ChatLoxP/LoxP and ChatLoxP/LoxP;Villin-CreERT2 mice. All mice were treated daily with tamoxifen for 5 days and analyzed 5 weeks later (Figure 2A). Chat Exon 8 sequence was amplified with genomic DNA from ChatLoxP/LoxP mice-enriched epithelial cells but was undetectable in ChatLoxP/LoxP;Villin-CreERT2 mice. Simultaneous amplification of the Villin-CreERT2 sequence confirmed the presence of the Villin-CreERT2 transgene uniquely in ChatLoxP/LoxP;Villin-CreERT2 mice as compared to ChatLoxP/LoxP mice, as well as the integrity of the genomic DNA purified from ChatLoxP/LoxP;Villin-CreERT2 mice (Figure 2B). This indicated highly efficient recombination of the Chat locus by the Villin-CreERT2 transgene. Moreover, since the Villin gene promoter is active in all intestinal epithelial cells, including stem cells,
23
CreERT2 activation by tamoxifen causes permanent gene deletion in the intestinal epithelium of compound ChatLoxP/LoxP;Villin-CreERT2 mice. Indeed, exon 8 deletion was very stable in spite of the rapid renewal of the intestinal epithelial cells, as indicated by the absence of exon 8 sequence amplification from ChatLoxP/LoxP;Villin-CreERT2 mice intestinal epithelial cell genomic DNA five weeks after tamoxifen treatment (Figures 2A and 2B). Of note, Villin-1 and Chat expression was reported in subsets of small intestinal type 2 innate lymphoid cell (ILC2) and ILC3 immune cell populations (Immunological Genome Project [ImmGen] database). To exclude any confounding non-epithelial CreERT2 contribution in the analysis of the ChatLoxP/LoxP;Villin-CreERT2 mice, we investigated the expression of the Villin-CreERT2 transgene product—more precisely, its nuclear translocation upon tamoxifen treatment—in small intestinal lamina propria ILC1, ILC2, and ILC3 subsets. Following tamoxifen injection (Figure 2C), nuclear CreERT2 could be detected in all epithelial cells but neither in CD45+ immune cells, nor, more specifically, in Tbx21+, Gata3+, and Rorγt+ immune subsets (Figure 2D), confirming the absence of Villin-CreERT2 transgene expression in mouse ILC1, ILC2, and ILC3 subsets.
We then investigated the consequences of the epithelial Chat deficiency on the cellular composition of the gut mucosa. No gross alteration of the epithelial layer was perceptible following tamoxifen treatment of ChatLoxP/LoxP;Villin-CreERT2 mice as compared to ChatLoxP/LoxP control mice, as identical representations of epithelial cells from the goblet, Paneth, and enteroendocrine cell lineages were found in both mouse genotypes (Figure S1A). Moreover, the overall distribution of the immune subsets (T cells, B cells, and myeloid cell populations including monocytes, neutrophils, macrophages, and mast cells) within the hematopoietic cell compartment in the intestine (intraepithelial and lamina propria fractions), as well as in more distant peripheral lymphoid organs (spleen, mesenteric, and axillary/brachial/cervical lymph nodes), was equivalent between ChatLoxP/LoxP;Villin-CreERT2 and ChatLoxP/LoxP mice (Figures S1B and S1C). More specifically, no difference was observed in the CD4 and CD8 T cell subpopulation as well as in their activation and polarization status (Figure S1B). Similarly, the relative percentage of ILC1, ILC2, and ILC3 subsets was comparable in the lamina propria and intraepithelial populations of ChatLoxP/LoxP;Villin-CreERT2 and ChatLoxP/LoxP mice (Figure S1C). We then assessed the consequences of the ChAT deficiency during an infection with parasitic helminths. We first induced Chat gene deletion by tamoxifen treatment during five consecutive days. After five days of rest without tamoxifen, ChatLoxP/LoxP and ChatLoxP/LoxP;Villin-CreERT2 mice were infected by gavage with H. polygyrus L3, and infection parameters were analyzed from 10 to 40 days post-infection when adult worms are present in the gut lumen (Figure 3A). Although ChAT-deficient mice had no obvious phenotype, autopsy revealed increased numbers of adult worms in these mice as compared to ChatLoxP/LoxP control mice (Figure 3B). Greater numbers of live worms were mirrored by the numbers of eggs in the feces of infected mice, a dynamic readout of the type 2 immune response efficiency and worm persistence. Indeed, a significantly increased number of eggs was found in ChAT-deficient mice, as compared to ChatLoxP/LoxP littermates, between 10 days post-infection (when adult worms start to be present in the gut lumen) and 40 days post-infection (Figure 3C). Increased numbers of adult worms and fecal eggs in ChAT-deficient mice thus revealed an essential role of tuft cell-derived ACh for an efficient defense against helminth parasites.
ACh concentrations are increased in tuft cells during type 2 immune responses
We then assessed the actual ACh concentration in tuft cells by mass spectrometry using lysates of FACS-sorted epithelial tuft cell fractions. ACh concentrations were significantly more elevated in the EpCam+; Siglec-F+ tuft cell fractions of mice infected with H. polygyrus as compared to the tuft cell fractions from naive mice, although high variability of ACh concentrations was present in infected mice, likely reflecting difference in the infection efficiency (Figure 3D). Because this elevation occurred in cellular populations enriched in tuft cells, it likely reflected increased ACh synthesis per tuft cell in efficiently infected mice rather than a consequence of increased tuft cell numbers caused by the epithelial remodeling consequent to type 2 immune responses. As expected, ACh was not detected in the tuft cell fractions from Chat-deficient epithelial cells in ChatLoxP/LoxP;Villin-CreERT2 mice (Figure 3D). Thus, the basal tuft cell ACh biosynthesis rate significantly increased in the context of a type 2 immune response against H. polygyrus infection.
ChAT-deficient mice are able to mount a type 2 immune response
To understand the mechanisms leading to decreased parasite clearance in ChatLoxP/LoxP;Villin-CreERT2 mice, we assessed critical parameters of the type 2 immune response and subsequent epithelial remodeling in naive and H. polygyrus-infected mice. Quantification of type 2 immune responses such as numbers of Gata3+ ILC2s/T helper 2 (Th2) cells, epithelial tuft cells, and goblet cells, and expression of the Retnlβ peptide by small intestinal goblet cells indicated the presence of a strong type 2 immune response in both ChatLoxP/LoxP control mice and ChatLoxP/LoxP;Villin-CreERT2 mice, with some of these parameters being even higher in infected ACh-deficient mice, possibly due to the presence of higher numbers of worms (Figures 4A–4C ). To determine whether increased type 2 immunity parameters in infected ChAT-deficient mice were more likely caused by higher worm burden or directly consequent to the ChAT deficiency, we triggered a worm-independent activation of tuft cells and subsequent type 2 immune response by treating ChatLoxP/LoxP and ChatLoxP/LoxP;Villin-CreERT2 mice with succinate. Succinate is a known activator of tuft cells, the only intestinal epithelial cells expressing the succinate receptor 1 (Sucnr1) and subsequent type 2 immune responses.
24
,
25
Nearly identical type 2 immune responses, as assessed by quantification of lamina propria Gata3+ cells, epithelial tuft cells, and Retnlβ expression in goblet cells were observed in both mouse groups (Figure S2). This suggested that the increased type 2 immunity parameters in H. polygyrus-infected ChatLoxP/LoxP;Villin-CreERT2 mice were more likely caused by an increased worm burden than a direct effect of the ChAT deficiency. We also assessed the mRNA expression of tuft cell mediators of type 2 immune responses and factors known to be involved in ACh synthesis and transport, including the Alox5, Alox5ap, Ltc4s, Ptgs1, Ptgs2, Hpgds, Pou2f3, Dclk1, Sucnr1, Il25, Chat, and VAChT genes using intestinal epithelial cell extracts isolated from ChatLoxP/LoxP and ChatLoxP/LoxP;Villin-CreERT2 in naive and 20 and 40 dpi mice infected with H. polygyrus. As expected, the Chat mRNA was absent in extracts from ChatLoxP/LoxP;Villin-CreERT2 mice. With the exception of the Dclk1 mRNA, which was found elevated in ChatLoxP/LoxP;Villin-CreERT2 mice at 40 days post-infection, no significant difference was found between control and ChAT-deficient mice at the naive or infected states (Figure S3). This was then confirmed at the cellular scale on tissue sections using immunofluorescence or in situ hybridization for all markers for which antibodies or probes were available (Alox5ap, Alox5, Ltc4s, Ptgs2, Hpgds, and Sucnr1). Again, no alteration in the expression patterns of these markers could be detected, suggesting that the ChAT deficiency did not alter substantially the expression of tuft cell alarmin molecules or their biosynthesis pathways (Figure S4), which is consistent with the observation of a strong type 2 immune response occurring in infected ChAT-deficient ChatLoxP/LoxP;Villin-CreERT2 mice.
To complement these data, we also sought to assess the distribution of the main immune cell populations present in the gut mucosa and distant lymphoid organs in control and ChAT-deficient infected mice. Unfortunately, the low viability of the cells dissociated from the inflamed gut mucosa of infected mice precluded such analyses. However, immunophenotyping could be performed on distant peripheral lymphoid organs (mesenteric lymph nodes, axillary/brachial/cervical lymph nodes, and spleen), and no significant differences were detected in ChAT-deficient infected mice as compared to controls (Figure S5 and STAR Methods). This suggested that the effects of tuft cell ACh deficiency remained local and did not affect distant or local lymphoid structures.
Mast cells constitute another immune subset involved in the defense against helminth parasites,
26
which can be analyzed by immunohistochemistry on inflamed tissue sections to circumvent the difficulty of studying dissociated cells from infected mice. We quantified the presence of mast cells in naive and infected mice using the mast cell protease 1 (Mcpt1) marker. In ChatLoxP/LoxP;Villin-CreERT2 mice, the Mcpt1+ cell population was more heterogeneous as compared to controls. Therefore, to quantify the difference in mast cell populations in control versus ChAT-deficient mice, we characterized the mast cell population detected in infected mice both in terms of percentage of the gut tissue with high density of mast cells and numbers of mast cells per microscopic field in regions of high mast cell densities (Videos S1, S2, S3, S4, S5, and S6). The rare Mcpt1+ cells found in naive ChatLoxP/LoxP mice strongly increased in H. polygyrus-infected mice 20 or 40 days post-infection. In ChAT-deficient mice, Mcpt1+ cell numbers also increased 20 days post infection but to a lesser extent compared to control mice, and at 40 days post infection, both ChatLoxP/LoxP and ChatLoxP/LoxP;Villin-CreERT2 mice had similarly elevated Mcpt1+ mast cell counts (Figures 5A–5C ). In both genotypes, Mcpt1+ mast cells almost always co-expressed other mast cell markers such as CD117 (mostly referred to as cKit and Granzyme B [GzmB]), as well as CD63, suggesting an activated state (Figures 5D and S6A). Moreover, quantification of Mcpt1+ cells co-expressing CD63 revealed similar rates of mast cell activation between ChatLoxP/LoxP and ChatLoxP/LoxP;Villin-CreERT2 mice, with 92.9% and 97.3% double-positive cells at 20 dpi and 91.2% and 92.4% at 40 dpi, respectively (Figure S6B). Together, these data indicated that ChatLoxP/LoxP;Villin-CreERT2 ACh-deficient mice were able to mount a strong type 2 immune response in the absence of tuft cell-derived ACh, with the exception of mast cells, which were transiently less numerous at 20 days post-infection in ChAT-deficient mice. Thus, higher worm persistence and egg production in ChAT-deficient mice were not due to a globally compromised type 2 immune response, indicating a yet unappreciated role of ACh in promoting worm expulsion.
Luminal ACh concentrations are increased following H. polygyrus infection
Certain helminth parasites express secreted isoforms of the AChE enzyme,
27
suggesting that degrading neighboring ACh is beneficial for the worms. In order to investigate the hypothesis of a direct effect of tuft cell-derived ACh on worms present in the host gut lumen, we next assessed luminal ACh concentrations in naive and H. polygyrus-infected mice. For this, an intestinal loop was surgically ligatured and filled with wash buffer, which was recovered after 30 min of incubation and processed for detection of ACh by mass spectrometry. The resulting measurements revealed significantly more elevated ACh concentration in the gut lumen of control-infected mice compared to naive animals (Figure 6A), which was likely due to contribution of both higher tuft cell numbers and increased ACh production per tuft cell, as indicated by ACh quantification from extracts of FACS-sorted tuft cells as compared to identical numbers of non-tuft intestinal epithelial cells (Figure 3D). We noted that ACh concentrations were variable in infected mice, probably reflecting infection efficiencies as well as the specific location of worms as regards the favorable intestinal loop for surgery. We then asked whether the actual presence of parasites was required for increased luminal ACh concentrations or whether this augmentation was directly linked to tuft cell activation. We thus treated mice with succinate and assessed ACh concentration changes. Indeed, luminal ACh was also significantly increased in the gut lumen of succinate-treated wild-type mice as compared to untreated mice, indicating luminal ACh release by tuft cells activated in the absence of worms (Figure 6B). Together, these data demonstrate the presence of tuft cell-derived ACh in the gut lumen of H. polygyrus-infected mice and suggest the possibility of a direct impact of luminal ACh on worm physiology.
Increased worm fitness in mice with ACh-deficient tuft cells
To directly assess the consequences of H. polygyrus exposure to ACh, we used two complementary approaches. Noteworthily, intestinal goblet cells produce the Retnlβ molecule, specifically in the context of type 2 immune responses, and this directly interferes with H. polygyrus physiology.
4
Subsequently, it was found that worms exposed to Retnlβ had decreased ATP concentrations, reflecting altered viability and fecundity as compared to unexposed worms.
5
,
6
Thus, using a similar approach, we quantified ATP concentrations in worms recovered from the intestines of ChatLoxP/LoxP and ChatLoxP/LoxP;Villin-CreERT2 mice as a proxy of their global fitness. No significant difference was found in ATP concentrations of worms from mice of either genotype recovered at 20 days post-infection. In contrast, by day 40, significantly increased ATP concentrations were found in worms from mice with ACh-deficient tuft cells as compared to control ChatLoxP/LoxP littermates, suggesting decreased fitness of the worms obtained from an environment containing ACh for several weeks (Figure 6C).
ACh exposure directly decreases worm fecundity
To confirm this finding, we also assessed worm fecundity ex vivo in the presence of 10 μM ACh, which is consistent with the micromolar range ACh concentrations found in the intestinal lumen lavage fluid obtained from H. polygyrus-infected ChatLoxP/LoxP control mice. Of note, it should also be taken into account the certainly partial recovery of luminal ACh using a single 30 min lavage as well as an estimated 15 times dilution factor corresponding to the lavage fluid volume injected into the intestinal loop (Figure S7). We thus quantified egg production per H. polygyrus adult female worm, freshly recovered from infected ChatLoxP/LoxP mice 14 days post-infection to ensure that the worms are present in the gut lumen and not yet strongly altered by luminal ACh. When ACh was added to the culture medium, egg production was significantly decreased as compared to untreated worms (Figure 6D). These data indicate that worm exposure to physiologically relevant ACh concentrations directly decrease helminth fecundity.
ACh targets worm physiology via their muscarinic ACh receptors
To identify the mechanisms underlying the effect of ACh on worm physiology, we assessed worm fecundity
5
,
6
in the presence of ACh combined with different inhibitors of cholinergic signaling. As shown previously in Figure 6D, exposure to ACh alone decreased worm fecundity as compared to untreated worms. Exposure to ACh combined with mecamylamine, an antagonist of the nicotinic AChRs, caused a comparable significant drop in worm fecundity. In contrast, the inhibitory effect of ACh on egg production was not observed with worms treated with a combination of ACh and atropine, an antagonist of the muscarinic AChRs (Figure 6E). This implies that the inhibitory effects of ACh on worm fecundity were mediated by muscarinic AChRs expressed by the worms. Finally, none of the drugs had significant effects on egg production when used alone (Figure 6E). Altogether, these data argue that in addition to their sentinel function in initiating type 2 immune responses, intestinal tuft cells act as effectors of such responses by releasing into the host lumen non-neuronal ACh in a range of concentrations capable of directly altering helminth fecundity through their muscarinic AChRs as assessed in mechanistic ex vivo experiments (Figure 7). In addition, luminally or basolaterally secreted ACh likely contributes to the increased presence of activated mast cells following H. polygyrus infection.
Discussion
Thus far, the importance of tuft cell-produced ACh has been mostly studied in the airways. In the mouse tracheal epithelium, tuft cells are capable of sensing bitter compounds present in the airway lining fluid and use cholinergic signaling to neighboring nerve endings to cause an aversive reflex consisting of a reduced breathing frequency.
10
Tuft cells also participate in the regulation of bacterial populations present in the airway. These cells can be activated by bacterial quorum sensing molecules (QSMs)—used by microbes to evaluate their own population density—such as 3-OxoC12-HLS and use ACh signaling to cause respiratory changes.
16
In addition to respiratory reflexes, mucociliary clearance is an important innate protective process to eliminate inhaled pathogens from the airway. Paracrine cholinergic signaling from tracheal tuft cells—activated by bitter compounds, QSMs, or other bacteria-derived products—was found to increase mucociliary clearance by airway ciliated cells, as assessed by the transport speed of particles on the tracheal surface, thereby directly linking chemosensation of bacterial signals with innate defense.
14
,
17
Previous studies with reporter mice also reported expression of Chat
11
and of the Tas2r143 bitter taste receptor
29
in a subset of tuft cells from the urogenital tract as well as from the gastrointestinal tract.
12
Furthermore, intraurethral application of a bitter compound regulated bladder activity in rats.
11
We confirmed intestinal tuft cell-restricted expression of the Chat gene for biosynthesis of ACh. Previous studies using green fluorescent protein (GFP) reporter driven by the Chat locus reported heterogeneity in GFP expression in trachea and urethra villin-immunoreactive tuft cells.
10
,
11
Instead, we found co-expression of the endogenous Chat mRNA and tuft cell Dclk1 protein in nearly all analyzed cells, suggesting either different regulation of Chat expression in the tuft cells from distinct organs or incomplete detection of the Chat gene product expression in genetically engineered reporter mice.
We present here two facets of a cholinergic function of small intestinal tuft cells. Firstly, we identified a regulator role on mastocytosis, the specific impact of which on the dynamics of H. polygyrus remains currently difficult to delineate. Secondly, we reported an effector function of tuft cells through the production of intestinal luminal ACh that may have direct effects on helminth parasites. Indeed, we reported the presence of ACh in the gut lumen with increased concentrations during type 2 immune responses in H. polygyrus-infected mice, reaching a micromolar range. Moreover, the increase in luminal ACh concentration could also be triggered by succinate treatment-driven tuft cell activation, suggesting that luminal ACh production is an integral component of the type 2 immune response downstream to tuft cell activation. Tuft cell-derived ACh directly or indirectly decreased worm viability 40 days post-infection, but not 20 days post-infection, as assessed by worm global ATP levels. Moreover, we demonstrated ex vivo a direct effect on worm fecundity following ACh exposure during 24 h, acting through H. polygyrus muscarinic AChRs. It is possible that in vivo quantification of global worm ATP concentrations, on the one hand, and ex vivo egg production, on the other hand, reflect ACh-dependent alterations of distinct physiological functions. Consistent with this, endogenous worm-derived ACh was reported to inhibit the egg laying behavior through worm muscarinic AChRs in the Caenorhabditis elegans helminth.
30
It is therefore plausible that host-derived ACh directly interferes with a related H. polygyrus muscarinic AChR pathway to decrease its fecundity. The reduced worm fitness observed 40 days post-infection might, in turn, rely on the alteration of distinct pathways, possibly involving nicotinic AChRs and related functions such as neuromuscular activity and feeding.
31
Luminal ACh released by tuft cells in the context of a type 2 immune response illustrates how different epithelial subsets constitute integral parts of this process. Signaling through epithelial IL-4Rα receptors causes specific amplification of two epithelial cell lineages, tuft cells and goblet cells. In addition to the effector function of tuft cells discussed above, goblet cells not only contribute to the so-called weep and sweep response by increased mucus production, they also express de novo the Retnlβ peptide that directly interacts with the helminth’s ability to feed on host tissues and thus also contribute to decreasing their fitness and fecundity.
4
,
5
,
6
As parasitic helminths have co-evolved with their hosts, it is plausible that the use of different defense mechanisms relying on distinct host cell types such as ACh and Retnlβ, produced by tuft and goblet cells, respectively, renders the task more challenging for parasites to counteract host immunity. In this view, it might be interesting to combine both ACh and Retnlβ host deficiencies to investigate possible synergistic action of these two molecules on different helminth parasite species.
Cholinergic regulation of immune responses is well described,
32
including in the context of helminth infections.
33
,
34
,
35
,
36
In the current study, we did not investigate the consequences of tuft cell ACh deficiency on immune subsets in more detail, with the exception of intestinal mucosal mast cells. This limited exploration was justified, because despite a strong type 2 immune response, no additional alterations in the expression of tuft cell-derived immune mediators, nor significant alterations of immune populations, were identified in ChatLoxP/LoxP;Villin-CreERT2 mice. We, however, reported that the mastocytosis observed following H. polygyrus infection was partially inhibited in mice with ChAT-deficient tuft cells. Although the precise links between mast cells and type 2 immune responses remain only partially understood, multiple studies reported decreased anti-parasitic defense in mouse lines with mast cell-deficiencies, including after infection with H. polygyrus.
37
,
38
Thus, although mastocytosis following helminth infection occurred in the absence of tuft cell-derived ACh, it was quantitatively lower as compared to infected control mice at 20 days post-infection, which might contribute to the delayed control of H. polygyrus infection observed in ChatLoxP/LoxP;Villin-CreERT2 mice. Noteworthily, several studies on the role of mast cells during helminth infections used cKit gene loss of function models
37
,
39
,
40
or its pharmacological inhibition
38
,
41
to cause mast cell deficiencies. cKit is also expressed in hematopoietic progenitors,
42
as well as in epithelial Paneth,
43
goblet,
44
and tuft cells,
45
which might be confounding if a tuft cell deficiency was induced beside the mast cell deficiency.
Luminal release of ACh and fine-tuning of mucosal mastocytosis may not be the sole modes of action of tuft cell ACh in the small intestine. Additional cholinergic mechanisms, such as paracrine signaling to other epithelial cells or nerve cells, as reported in the airway,
10
,
14
were recently reported in the small intestine.
28
Moreover, additional ACh sources, such as ILC2s, exist in the gut mucosa and also contribute to ILC2 numbers inflation and anti-helminth immunity.
35
Our study on the cholinergic functions of intestinal tuft cells thus revealed additional functions for these cells with a luminal mode of action of ACh. Hence, we propose that tuft cells play two distinct but complementary roles in the context of the host defense against helminth infections. In the naive mucosa, tuft cells are a rare epithelial subset functioning as a sentinel to initiate type 2 immune responses upon parasitic infections. Our findings now document how the amplification of the tuft cell lineage, occurring in the context of an ongoing type 2 immune response, underlies a second function as a cholinergic regulator of mastocytosis and effector that likely directly contributes to parasitic helminth clearance by compromising their global fitness. Moreover, as demonstrated ex vivo, ACh concentrations measured in the gut lumen can directly alter H. polygyrus fecundity. This not only highlights the role of tuft cell hyperplasia during type 2 immune responses but may also provide a missing link to understand why IL-25 deficiency, a key tuft cell cytokine involved in their sentinel function,
8
causes a less severe phenotype than the complete absence of tuft cells in Pou2f3-deficient mice.
12
Limitations of the study
Despite these insights, several questions remain. First, the tuft cell mechanisms underlying ACh secretion remain unclear. A non-canonical pathway might be involved since previous expression studies on the VAChT protein, required for the uptake of ACh into small synaptic vesicles, and the high-affinity choline transporter (ChT1), required for the re-uptake of choline to promote intracellular ACh synthesis, reported the absence of detectable expression of these two proteins in tuft cells from all intestinal segments.
12
Second, our study introduces the notion that tuft cells release ACh as a molecule that directly targets helminth parasites. This notion might be expanded with the discovery of additional tuft cell factors contributing to the same function against helminths or other intestinal parasites. Finally, future studies will be needed to evaluate the potential therapeutic perspectives consecutive to our findings in refining the common anti-helminth treatments of humans or livestock, such as cholinergic agonists or inhibitors of AChEs, with a focus on a luminal mode of action of these drugs.
STAR★Methods
Key resources table
REAGENT or RESOURCESOURCEIDENTIFIERAntibodiesRabbit polyclonal anti Dclk1AbcamCat# Ab31704; RRID: AB_873537Sheep polyclonal to Dclk1R&D SystemsCat# AF7138; RRID: AB_10973467Rabbit monoclonal [EPR16651] to Gata3AbcamCat# Ab199428; RRID: AB_2819013Rabbit polyclonal to RetnlbAntibodies onlineCat# ABIN465494Mouse monoclonal to Insm1Santa CruzCat# sc-271408; RRID: AB_10607955Rabbit polyclonal to LysozymeDakoCat# A0099; RRID: AB_2341230Rabbit polyclonal to Granzyme-B antibodyAbcamCat# Ab4059; RRID: AB_304251Rat monoclonal to Cd45NovusCat# NB100-77417; RRID: AB_1083776Rat monoclonal to MCPT-1 (mMCP-1) AntibodyeBioscience/ThermoFisherCat# 14-5503-82; RRID: AB_10854869Goat polyclonal to C-Kit antibodyR&D SystemsCat# AF1356; RRID: AB_354750Rabbit polyclonal to Collagen V antibodyGift From Patricia Simon AssmannN/ARat anti mouse Siglec-F, PE-conjugatedBD pharmigenCat# 552126; RRID: AB_394341Rat anti mouse EpCam, APC-conjugatedeBioscience/ThermoFisherCat# 17-5791-82;
RRID: AB_2716944Rabbit monoclonal to 5-Lipoxygenase Antibody (5Alox)NovusCat# NBP3-161-50Goat polyclonal to FLAP (Alox5Ap) AntibodyNovusCat# NB300-891; RRID: AB_2227081Rabbit polyclonal to cyclooxygenase 2 antibodyAbcamCat# Ab225273Rabbit polyclonal to Prostaglandin D Synthase (hematopoietic-type) AntibodyCayman chemicalCat# 160013;
RRID: AB_10080037Rabbit monoclonal to CD63 antibodyAbcamCat# Ab217345;
RRID: AB_2754982Mouse monoclonal to Estrogen Receptor alpha antibodySanta CruzCat# Sc-8002;
RRID: AB_627558Rabbit Monoclonal Anti-TBX21 (T-bet)ClinisciencesCat# AC-0240ARabbit Anti-Rorgt (clone EPR20006)AbcamCat# ab207082; RRID: AB_2889310Rat Anti-mCD45-FITC (clone 30-F11)BD BiosciencesCat# 553080; RRID: AB_394610Rat Anti-mCD45-APC (clone 30-F11)BD BiosciencesCat# 559864; RRID: AB_398672Rat Anti-mCD45- APC-eF780 (clone 30-F11)eBioscience/ThermoFisherCat#47-0451-80;
RRID: AB_1548790Rat Anti-mCD19- BV650 (clone 1D3)BD BiosciencesCat# 563235;
RRID: AB_273808Rat Anti-mCD19- V450 (clone 1D3)BD BiosciencesCat# 560375;
RRID: AB_164526Hamster Anti-CD11c- PE (clone HL3)BD BiosciencesCat#557401;
RRID: AB_396684Rat Anti-mCD11b-BV605 (clone M1/70)BD BiosciencesCat# 563015; RRID: AB_2737951Rat Anti-mCD4- BV711 (clone RM4-5)BD BiosciencesCat#563726;
RRID: AB_2738389Rat Anti-mCD4- BV786 (clone RM4-5)BD BiosciencesCat# 563727;
RRID: AB_2728707Hamster Anti-mTCRβ- FITC (clone H57-597)BD BiosciencesCat# 553170; RRID: AB_394682Hamster Anti-mTCRβ- AF594 (clone H57-597)BiolegendCat# 109238;
RRID: AB_2563324Hamster Anti-mTCRβ- V450 (clone H57-597)BD BiosciencesCat# 560706; RRID: AB_1727576Rat Anti-mCD8α- APC-R700 (clone 53-6.7)BD BiosciencesCat#564983;
RRID: AB_2739032Rat Anti-mCD8b- PE (clone H35–17.2)BD BiosciencesCat# 550798; RRID: AB_393887Rat Anti-mCD117- BV711 (clone2B8)BD BiosciencesCat# 563160; RRID: AB_272251Rat Anti-mCD117- PECF594 (clone2B8)BD BiosciencesCat# 562417; RRID: AB_11154233Rat Anti-mSca-1- FITC (clone D7)BD BiosciencesCat# 557405;
RRID: AB_396688Rat Anti-mCD127- PE (clone A7R34)eBioscience/ThermoFisherCat# 12-1271-82; RRID: AB_465844Hamster Anti-mKLRG1- PeCy7 (clone 2F1)eBioscience/ThermoFisherCat# 25-5893-82; RRID: AB_1518768Anti-mGATA3- V450 (clone L50-823)BD BiosciencesCat# 563349;
RRID: AB_2738152Mouse Anti-mRoRγt- BV650 (clone Q31378)BD BiosciencesCat# 564722; RRID: AB_2738915Mouse Anti-mTbet- PE (clone 4B10)eBioscience/ThermoFisherCat#12-5825-82;
RRID: AB_925761Rat Anti-mF4/80- PeCy7 (clone BM8)eBioscience/ThermoFisherCat# 25-4801-82;
RRID: AB_469653Rat Anti-mLy6C- V450 (clone HK1.4)eBioscience/ThermoFisherCat# 48-5932-82; RRID: AB_10805519Rat Anti-mLy6G- PE-CF594 (clone 1A8)BD BiosciencesCat# 562700; RRID: AB_2737730Hamster Anti-mFceRIα - APC (clone MAR-1)eBioscience/ThermoFisherCat# 17-5898-80;
RRID: AB_10717073Live/Dead V506eBioscience/ThermoFisherCat#65-0866-14Donkey Anti-Rabbit IgG H&L (Alexa Fluor® 555)AbcamCat# ab150074;
RRID: AB_2715537Donkey Anti-Rabbit IgG H&L (Alexa Fluor® 647)AbcamCat# ab150075;
RRID: AB_2752244Donkey Anti-Rat IgG H&L (Alexa Fluor® 647) preadsorbedAbcamCat# ab150155;
RRID: AB_2813835Donkey Anti-Rat IgG H&L (Alexa Fluor® 488) preadsorbedAbcamCat# ab150153;
RRID: AB_2737355Donkey Anti-Mouse IgG H&L (Alexa Fluor® 555)AbcamCat# ab150106;
RRID: AB_2857373Donkey Anti-Mouse IgG H&L (Alexa Fluor® 488)AbcamCat# ab150105;
RRID: AB_2732856Donkey Anti-Sheep IgG H&L (Alexa Fluor® 488)AbcamCat# ab150177;
RRID: AB_2801320Donkey Anti-Goat IgG H&L (Alexa Fluor® 555)AbcamCat# ab150130;
RRID: AB_2715537Chemicals, peptides, and recombinant proteinsNeostigmine BromideSigma-AldrichCat# N2001Acetylcholine chlorideSigma-AldrichCat# A6625AtropineSigma-AldrichCat# A0132Mecamylamine hydrochlorideSigma-AldrichM9020RPMI culture mediumLife TechnologiesCat# 21875-034DMEM culture mediumLife TechnologiesCat# 21969-035FBSSigma-AldrichCat# F7524DispaseCorningCat# 354235DNAse 1Sigma-AldrichCat# 112849320017-aminoactinomycin DLife TechnologiesCat# A1310HBSS 10X w/o Ca2+/Mg2+GIBCOCat# 14185-045HBSS 1X avec Ca2+/Mg2+GIBCOCat# 14025-050PBS 1X (Ca2+ et Mg2+ free)EurobioCat# CS1PB01-01LiberaseTMRocheCat# 5401119001EDTASigma-AldrichCat# 03690-100DTTSigma-AldrichCat# 43816-50Vetflurane 1000 mg/gVirbacGTIN 03597132002653Buprecare 0.3 mg/mlAxienceGTIN 03760087151244TamoxifeneSigma-AldrichCat# T5648Laocaine 16mgMSDGTIN: 05017363520132Trizol reagentThermo FisherCat# 15596026BuprenorphineSigma-AldrichCat#B9275Hematoxylin Solution, Gill No. 3Sigma-AldrichCat#GHS316SIGMAFAST™ 3,3′-Diaminobenzidine tabletsSigma-AldrichCat# D4293N-HistofineNichirei Biosciences414151FCritical commercial assaysATPLite kitPerkin ElmerCat# 6016943RNeasy Micro KitQiagenID: 74004RNeasy Mini KitQiagenID: 74104Transcriptor First Strand cDNA Synthesis KitRoche lifescienceCat# 04896866001LightCycler® 480 SYBR Green I MasterRoche lifescienceCat# 04887352001RNAscope™ Multiplex Fluorescent V2 AssayACD a biotechne brandCat# 323270Fix/perm kit FOXP3/transcription factor staining buffer seteBioscience/ThermoFisherCat# 00-5523-00Arcturus RiboAmp Plus kitThermo FisherCat# KIT0501Experimental models: Organisms/strainsMouse strain Chattm1MltLecomte et al.
22
Provided by S. BerrardMouse strain Tg(Vil1-cre/ERT2)23SyrEl Marjour et al.
23
Provided by S. RobineMouse strain ChatLoxP/LoxP; VillinCreERT2/+This studyN/AHeligmosomoides polygyrusProvided by R. MaizelsProvided by R. MaizelsOligonucleotidesChatForward TTTGCAGCCAGCCTCATCTC
Reverse TATGGCCAGGAAGCCGGTATEurofinsVAChTForward GGCCTCGCTACCCCACAGAA
Reverse CCCAGGCCAATAAGCAGCGGEurofinsPou2f3Forward GAGGGAATGATGAGCCCACT
Reverse GTGAAGCCTAGCTTAATGCGTCEurofinsAlox5Forward TCTTCCTGGCACGACTTTGCTG
Reverse GCAGCCATTCAGGAACTGGTAGEurofinsAlox5apForward GTTCTTTGCCCACAAGGTGGAG
Reverse TGCAGTCCAGAGTACCACAAGGEurofinsPtgs1Forward GAATGCCACCTTCATCCGAGAAG
Reverse GCTCACATTGGAGAAGGACTCCEurofinsPtgs2Forward TCAATGAGTACCGCAAACGC
Reverse AGGGTACAGTTCCATGACATCGEurofinsHpgdsForward GAATAGAACAAGCTGACTGGC
Reverse AGCCAAATCTGTGTTTTTGGEurofinsLtc4sForward CCTACAGGTGATCTCTGCACGA
Reverse TGGCGAGGAACAGCGGAAAGTAEurofinsSucnr1Forward CCATCTCTGACTTTGCTTTCCTG
Reverse GTGTAGAGGTTGGTGTGAAGCACEurofinsDclk1Forward CAGCCTGGACGAGCTGGTGG
Reverse TGACCAGTTGGGGTTCACATEurofinsIl25Forward CCTGTCAGGCAGGGGTAGTA
Reverse CCAAGAATGCAACAGCCTGEurofinsVillinForward: CAAGCCTGGCTCGACGGCC
Reverse: CGCGAACATCTTCAGGTTCTEurofinsChat (exon8)Forward: CCCGTTTTCCTTCCAGCCAA
Reverse: CGCGAACATCTTCAGGTTCTEurofinsGapdhForward GGAGCGAGACCCCACTAACA
Reverse ACATACTCAGCACCGGCCTCEurofinsHprtForward GCAGTACAGCCCCAAAATGG
Reverse GGTCCTTTTCACCAGCAAGCTEurofinsSoftware and algorithmsNdpView.V2Hamamatsu Photonicshttps://www.hamamatsu.comZen blue edition. V3.2Zeisshttps://www.zeiss.com/microscopy/fr/produits/logiciel/zeiss-zen.htmlPhotoshop CS6. V13.0Adobehttps://www.adobe.com/fr/products/photoshop.htmlPrism10.1GraphPadhttps://www.graphpad.comLightCycler 480 V1.5Rochehttps://lightcycler-software.software.informer.comImageJ. V1.8Wayne Rasband (NIH)https://imagej.net/ij/download.htmlFlowJo, LLC V9Tree Star; Ashland, ORhttps://www.flowjo.com/BiorenderBioRENDERhttps://www.biorender.com/OtherRNAscope™ Probe- Mm-Chat-C2Advanced Cell DiagnosticsCat# 408731-C2RNAscope™ Probe- Mm-Sucnr1-C3Advanced Cell DiagnosticsCat# 437721-C3RNAscope™ Probe- Mm-Ltc4s-C3Advanced Cell DiagnosticsCat# 1046751-C3
Resource availability
Lead contact
Further information and requests for resources and reagents should be directed to and will be fulfilled by the lead contact, Philippe Jay (philippe.jay@igf.cnrs.fr).
Materials availability
All stable reagents generated in this study are available from the lead contact with a completed materials transfer agreement.
Data and code availability
•
All data reported in this paper will be shared by the lead contact upon request.
•
This paper does not report original code.
•
Any additional information required to reanalyze the data reported in this paper is available from the lead contact upon request.
Experimental model and study participant details
Mouse models
The conditional ChatLoxP/LoxP allele, (Chattm1Mlt,
22
) was kindly provided by Sylvie Berrard and crossed with the intestinal epithelium-specific, tamoxifen-inducible, Villin-CreERT2 mouse strain (Tg(Vil1-cre/ERT2)23Syr.
23
All the mice were bred and maintained in an SOPF animal facility. All animal experiments were conducted in accordance with the French Ministry for Education and Research, regarding the care and use of animals for experimental procedures, under the 2022020111554986, and 2019031411197134 Apafis references, according the ARRIVE Guidelines. Male and female mice were analyzed between 8 and 12 weeks of age. Cohorts of controls and deficient mice were obtained from littermates. No statistical method was used to predetermine sample size and the experiments were not randomized. Unless otherwise stated, the investigators were not blinded to allocation during experiments and outcome assessment.
Method details
Animal procedures
Cre-mediated recombination was achieved with a daily intraperitoneal injection of 1 mg of tamoxifen (Sigma), for 5 consecutive days. For H. polygyrus infection experiments, mice were inoculated orally with 200 infective L3. Infection parameters were monitored according to the numbers of fecal eggs, or numbers of intestinal adult worms from day 10 to day 40-post infection. Samples of luminal intestinal contents were obtained from naive or infected mice, according to the following surgery procedure. After 4 h of fasting, mice received subcutaneously a single dose of buprenorphine (0.1 mg/kg body weight), and were anesthetized 30 min later with 3% isoflurane. After local lidocaine treatment, the abdominal cavity was opened, and an intestinal loop was ligatured. This intestinal loop was filled with up to 400 μL of a PBS wash solution containing 5 μM of Neostigmine Bromide (Sigma). The loop content was recovered 30 min later, after which mice were euthanized.
Collection of adults H. polygyrus and in vitro culture for treatment with cholinergic drugs
Adult H. polygyrus worms were collected from the intestinal lumen of infected mice at 14 dpi to evaluate ex vivo the effects of cholinergic drugs on egg release. Worms were washed with PBS containing penicillin (5U/ml)/streptomycin (5 μg/ml)/gentamicin (1%), counted, and 20 to 25 female worms per well were incubated at 37°C, 5% CO2, in 200μL RPMI containing antibiotics. Adult H. polygyrus worms were then treated with the following drugs: acetylcholine chloride (Sigma, A6625) at 10μM, atropine (Sigma, A0132), and mecamylamine hydrochloride (Sigma, M9020) at 5μM. After 24h, culture media were mixed with a saturated NaCl solution (in a 50/50 volume ratio) to count the eggs. For global worm fitness evaluation, worms were collected from mice at 20 and 40 dpi, washed in PBS, and directly used as substrate for ATP quantification, using the ATPLite kit (6016943; PerkinElmer), according to the manufacturer’s instructions.
Cell sorting experiments
Single intestinal epithelial cells were obtained from small intestines after incubation in ice-cold 30mM EDTA (Sigma) in HBSS pH 7.4 (Life Technologies) for 20 min. Tissues were then vigorously shaken in DMEM (Life Technologies) supplemented with 10% FBS (Sigma), with 100μL of Dispase (Corning), and 100μL of DNase I at 2,000 Kunitz (Sigma). After filtration on a 40 μm mesh, single cell suspensions were incubated with phycoerythrin rat anti-mouse Siglec-F antibody (BD Pharmigen, 552126), and FITC rat anti mouse EpCam antibody (17-5791-82, ebiosciences) for 30 min at 4°C, and washed with HBSS and resuspended in appropriate volume of HBSS pH 7.4 supplemented with 5% FBS before staining with 7-aminoactinomycin D (Life Technologies) to exclude dead cells. Siglec-F+ live cells were sorted using a FACSAria (Becton Dickinson), directly in RLT lysis buffer (Qiagen) for subsequent RNA extraction, or methanol for ACh quantification assays. After removing epithelial cell fractions as described above, the remaining tissues were proceeded for lamina propria cell preparation. Tissues were washed twice with RPMI 1640 (Life technologies) supplemented with 5% FCS, and minced into small pieces and digested with a solution composed with RPMI 1640, 100μg/mL of Liberase TM (Roche) and 50μg/mL of DNase I (Sigma-Aldrich) for 30 min at 37°C under gentle shaking. Supernatants were filtered onto a 40μm mesh. After a 5 min centrifugation at 2000RPM, 4°C, cell pellets were resuspended in 500μL PBS-5% FCS-2mM EDTA for further stainings.
Immunophenotyping and flow cytometric analysis
Cells isolated from peripheral lymph nodes, mesenteric lymph nodes, spleen and intestinal tissue (lamina propria and intra-epithelial lymphocytes) were stained with Live/dead fixable viability dye (Ebioscience/Thermofisher) together with the appropriate conjugated anti-TCRβ, CD45, CD19, CD4, CD8, CD11b, Ly6G, Ly6C, FcεRIa, F4/80, CD117 (eBioscience/Thermofisher or Becton Dickinson) at a 1:200 dilution (mAb), in a total volume of 50–100 μls as previously described. Cells were incubated in the dark for 20 min in PBS containing 2% FBS at 4°C and then washed once in the same medium at 300 g for 5 min prior to be evaluated by flow cytometry. For ILC staining, cells were stained with a lineage cocktail and lineage-negative CD45+ cells were assessed for expression of CD127, and intracellular expression of Gata-3 (clone L50-823), RoRγt and Tbx21 was performed following fixation/permeabilization (eBioscience/Thermofisher). Stained cells were assessed by flow cytometry (LSR Fortessa, Becton Dickinson, San Jose, CA) and a minimum of 10,000 events were recorded for each staining. Data analyses were performed using FlowJo software (Tree Star, Ashland, OR).
ACh quantification
Known numbers of sorted tuft cells (recovered in pure methanol) and intestinal lavage samples (0.2 mL, immediately mixed with 0.8 mL pure methanol), were kept at −80C° for 48h to allow protein precipitation. Next, 2-morpholinoethansulfonic acid (Cat. # 341–01622; Dojindo, Tokyo, Japan) was added as quality control (QC) standard at 0.5 μM final concentration (FC) and the samples were then supplemented with formic acid to 1% FC. The samples were vortexed vigorously for 1 min and then centrifuged for 10 min at 20.000xg to precipitate proteins. A volume of 0.9 mL supernatant was then loaded on the Captiva EMR plate (Agilent, Santa Clara, USA, Cat.# 5190-1001) assembled on the Vacuum Manifold (Agilent, Cat.# A796) together with the Deep Well collection plate (Agilent, Cat.# A696001000). The flow-through fractions were first dried using a Speedvac concentrator (Thermo Scientific, Cat.# SPD2030) and then resuspended in 200 μL of water (Biosolve BV, Cat. # 0023214102BS). Calibration curve was established by diluting defined amounts of ACh in the matrix (generated by intestinal lavage of KO mice). Protein quantities from each sample were measured using the BCA kit (Thermo Scientific) according to manufacturer’s recommendations. Briefly, protein pellets were solubilized in 0.3 mL of 2% sodium dodecyl sulfate solution (Sigma Aldrich, Cat. # 05030) in water. Two μL of sample was then used to estimate the protein content of each sample.
One microliter of resuspended Captiva flow-through fraction or 1 μL of standard were injected on LC-MS systems consisting of UHPLC (Agilent, 1290 Infinity II Biocompatible) coupled to triple quadrupole MS (Agilent, 6495C). The samples were analyzed using in MRMs acquisition mode. Following transitions were used to quantify ACh: 147.1 → 43.0, 147.1 → 88.1, 147.1 → 87.1 (positive mode). The transitions for the 2-morpholinoethansulfonic acid were: 196.2 → 100.0 (positive) and 194 → 80.15 (negative). The retention times were set as follows: 2.04 min and 8 min, for 2-morpholinoethansulfonic acid and ACh respectively. The analytical column was UPLC Discovery column HS F5-3 (Cat. # 567503-U; Sigma Aldrich). Mobile phases were composed as follows: A: 99.9% water (Biosolve BV, Cat. # 0023214102BS), 0.1% formic acid (Sigma Aldrich, Cat. # 33015); B: 99.9% acetonitrile (Biosolve BV, Cat.# 001204102BS), 0.1% formic acid. The gradient was: 0 min (100% A), 2 min (100% A), 5 min (75% A), 11 min (65% A), 15 min (5% A), 25 min (5% A), 25.10 min (100% A), 35 min (100% A). Flow rate was 0.25 mL/min and the temperature column was set to 40°C. The following source parameters were used: Gas Temperature: 150°C; Gas Flow: 11 L/min; Nebulizer: 40 psi; Sheath Gas Temperature: 400°C; Sheath Gas Flow: 12 L/min; Capillary Voltage (neg. mode): 4000 V; Capillary Voltage (pos. mode): 4000 V; Nozzle Voltage: 500 V; IFunnel High Pressure RF Pos: 100 V Neg: 50 V; IFunnel Low Pressure RF Pos: 100 V Neg: 50 V.
Following the analysis, peak integration was conducted using Agilent Masshunter Quantitative Analysis software (version 10.1.733.0). The absolute quantification of ACh was calculated as previously demonstrated, by applying the peak area from each sample to the ACh calibration curves.
46
RNA extraction and PCR
Total RNAs from intestinal tissues were isolated using TRIzol (Life Technologies). RNeasy Micro and Mini Kit columns (Qiagen) were also used for RNA purification from cell-sorted experiments. Sorted-cell RNA were further amplified using the Arcturus RiboAmp Plus kit (ThermoFischer Scientific, KIT0501) according to the manufacturer’s instructions. Reverse transcription was performed with 1 μg of purified RNA using Transcriptor First Strand cDNA synthesis KIT (Roche) according to the manufacturer’s instructions. Real-time quantification was performed in triplicate with a LightCycler480 (Roche) using LightCycler 480 SYBR Green I Master (Roche) on 5ng of RT product using the average Ct of Gapdh and Hprt as internal loading controls, and the ΔΔCt method was used for calculating relative expression.
Fluorescent immunohistochemistry or in situ hybridization on paraffin-embedded tissue
Tissue dissection, fixation, and immunohistochemistry on thin sections of paraffin-embedded tissue were performed essentially as described previously.
47
Epitope retrieval was achieved by boiling in 10 mM in sodium citrate (pH 6.4) during 20 min. Primary antibodies used in this study were incubated ON at 4°C, and are listed in the key resource table. Slides were then washed twice with 0.1% PBS-Tween (Sigma-Aldrich) before incubation with fluorescent dyes-conjugated secondary antibodies (Jackson ImmunoResearch Laboratories, Inc.) and DAPI at 2 μg/mL (Sigma-Aldrich) in TBS–Triton X-100 0.1% (Sigma-Aldrich), or HRP-conjugated secondary antibodies, revealed with DAB (Sigma). Slides were mounted in FluoroMount (Sigma) or Pertex (Histolab), for fluorescent or visible imaging, respectively. For mRNA in situ hybridisation, tissues were hybridised with a probe targeting the Chat mRNA (Cat No. 408731, ACDBio), SucnR1 (Cat No 437721), and Ltc4s (Cat No 1046751). Slides were processed according to the manufacturer’s instructions until probe revelation, after which immunofluorescence detection of Dclk1 was performed following the methodology described above.
Microscopy and imaging
Fluorescent pictures were acquired at room temperature on an AxioImager Z1 microscope (Carl Zeiss, Inc.) equipped with a camera (AxioCam MRm; Carl Zeiss, Inc.), EC Plan Neofluar (5X NA 0.16; 10X NA 0.3; 20X 0.5 NA; 100X NA 1.3) and Plan Apochromat (40X NA 0.95; 63X NA 1.4) lenses, apotome Slider system equipped with an H1 transmission grid (Carl Zeiss, Inc.), and Zen software (Carl Zeiss, Inc.). Post-treatment of pictures (level correction), annotations, and panel composition were performed using the Photoshop (Adobe) or Zen (Carl Zeiss) softwares. Bright-field immunohistochemistry pictures were taken at room temperature on an Eclipse 80i microscope (Nikon) with Plan Fluor (10X NA 0.3; 20X NA 0.5; 40X NA 0.75; and 60X NA 0.5–1.25) lenses (Nikon) and a digital camera (Q-Imaging Retiga 2000R with a Q-Imaging RGB Slider), with Q-Capture Pro software (Nikon). Stained slides were also imaged with a Nanozoomer device (Hamamatsu), visualised and annotated with the NdpView Software (Hamamatsu).
Quantification and statistical analyses
The Prism software was used for descriptive statistical analyses. After a normality distribution test, datasets were analyzed with either a two-tailed Student T test (normal distribution, pairwise comparisons) or with the non-parametric two-tailed Mann–Whitney U-test (nonnormal distribution, pairwise comparisons). For multiple comparisons, datasets were proceeded with one-way ANOVA analyses (normal distribution, with Benjamini-Yekutieli post-hoc correction) or with Kruskal-Wallis analyses (nonnormal distribution, with Benjamini-Yekutieli post-hoc correction). For some analyses, multiple Mann-Whitney analyses were performed. Depending on the normality distribution, datasets are represented in each graph as individual values and bars corresponding to mean ± SD (normal distribution) or either as individual values and bars corresponding to median ± interquartile (nonnormal distribution). Details for statistical analyses are mentioned in each figure legend.
Acknowledgments
We acknowledge Prof. M. Selkirk for scientific discussions, Dr. E. Valjent for technical inputs and reagents, Elisa Evain for help in immunophenotyping experiments, the RAM-iExplore and RAM-PCEA facilities for maintenance of mouse colonies, and the Montpellier RIO Imaging and Arpege facilities. This work was supported by a Wellcome Trust Collaborative Award (ref. 211814) to P.J., C.B., E.D., T.M., and R.M.; Agence Nationale de la Recherche (ANR-17-CE15-0016-01 and ANR-21-CE15-0017-01 to P.J.); Institut National Du Cancer (INCA_2018-158 to P.J.)—the P.J. team is “Equipe Labellisée Ligue contre le Cancer”; M.N. was supported by the Labex EpiGenMed (an “Investissements d’avenir” program ANR-10-LABX-12-01) and the Wellcome Trust Collaborative Award (ref. 211814). We acknowledge the French Ministry of Higher Education and Research for supporting the PLATON facility through a CPER grant (IBDLR) as well as Oriane Scholler (PLATON) for technical discussion.
Author contributions
Conceptualization, P.J., F.G., and R.M.M.; methodology, M.D., F.H., C.J., A.T., and S.T.; investigation, M.D., F.G., F.H., I.G., C.J., J.B., A.L., N.C., S.H., V.D., V.S.Z., M.C.P., E.T., A.G., and C.C.; writing—original draft, P.J. and F.G.; writing—review & editing, P.J., F.G., R.M.M., C.B., E.D., T.N.M., V.D., V.S.Z., A.T., and S.B.; funding acquisition, R.M.M., P.J., C.B., E.D., and T.N.M.; resources, S.B., Z.H., J.P., L.F., and C.C.; supervision, P.J., F.G., and R.M.M.
Declaration of interests
The authors declare no competing interests.
Supplemental information
References
Gerbe F.
Sidot E.
Smyth D.J.
Ohmoto M.
Matsumoto I.
Dardalhon V.
Cesses P.
Garnier L.
Pouzolles M.
Brulin B.
et al.
Intestinal epithelial tuft cells initiate type 2 mucosal immunity to helminth parasites.
Nature. 2016; 529: 226-230https://doi.org/10.1038/nature16527
View in Article
Howitt M.R.
Lavoie S.
Michaud M.
Blum A.M.
Tran S.V.
Weinstock J.V.
Gallini C.A.
Redding K.
Margolskee R.F.
Osborne L.C.
et al.
Tuft cells, taste-chemosensory cells, orchestrate parasite type 2 immunity in the gut.
Science. 2016; 351: 1329-1333https://doi.org/10.1126/science.aaf1648
View in Article
von Moltke J.
Ji M.
Liang H.-E.
Locksley R.M.
Tuft-cell-derived IL-25 regulates an intestinal ILC2–epithelial response circuit.
Nature. 2016; 529: 221-225https://doi.org/10.1038/nature16161
View in Article
Artis D.
Wang M.L.
Keilbaugh S.A.
He W.
Brenes M.
Swain G.P.
Knight P.A.
Donaldson D.D.
Lazar M.A.
Miller H.R.P.
et al.
RELMbeta/FIZZ2 is a goblet cell-specific immune-effector molecule in the gastrointestinal tract.
Proc. Natl. Acad. Sci. USA. 2004; 101: 13596-13600https://doi.org/10.1073/pnas.0404034101
View in Article
Herbert D.R.
Yang J.Q.
Hogan S.P.
Groschwitz K.
Khodoun M.
Munitz A.
Orekov T.
Perkins C.
Wang Q.
Brombacher F.
et al.
Intestinal epithelial cell secretion of RELM-beta protects against gastrointestinal worm infection.
J. Exp. Med. 2009; 206: 2947-2957https://doi.org/10.1084/jem.20091268
View in Article
Chen G.
Wang S.H.
Jang J.C.
Odegaard J.I.
Nair M.G.
Comparison of RELMα and RELMβ Single- and Double-Gene-Deficient Mice Reveals that RELMα Expression Dictates Inflammation and Worm Expulsion in Hookworm Infection.
Infect. Immun. 2016; 84: 1100-1111https://doi.org/10.1128/IAI.01479-15
View in Article
Molofsky A.B.
Locksley R.M.
The ins and outs of innate and adaptive type 2 immunity.
Immunity. 2023; 56: 704-722https://doi.org/10.1016/j.immuni.2023.03.014
View in Article
Fallon P.G.
Ballantyne S.J.
Mangan N.E.
Barlow J.L.
Dasvarma A.
Hewett D.R.
McIlgorm A.
Jolin H.E.
McKenzie A.N.J.
Identification of an interleukin (IL)-25-dependent cell population that provides IL-4, IL-5, and IL-13 at the onset of helminth expulsion.
J. Exp. Med. 2006; 203: 1105-1116https://doi.org/10.1084/jem.20051615
View in Article
Wessler I.
Kirkpatrick C.J.
Cholinergic signaling controls immune functions and promotes homeostasis.
Int. Immunopharmacol. 2020; 83106345https://doi.org/10.1016/j.intimp.2020.106345
View in Article
Krasteva G.
Canning B.J.
Hartmann P.
Veres T.Z.
Papadakis T.
Mühlfeld C.
Schliecker K.
Tallini Y.N.
Braun A.
Hackstein H.
et al.
Cholinergic chemosensory cells in the trachea regulate breathing.
Proc. Natl. Acad. Sci. USA. 2011; 108: 9478-9483https://doi.org/10.1073/pnas.1019418108
View in Article
Deckmann K.
Filipski K.
Krasteva-Christ G.
Fronius M.
Althaus M.
Rafiq A.
Papadakis T.
Renno L.
Jurastow I.
Wessels L.
et al.
Bitter triggers acetylcholine release from polymodal urethral chemosensory cells and bladder reflexes.
Proc. Natl. Acad. Sci. USA. 2014; 111: 8287-8292https://doi.org/10.1073/pnas.1402436111
View in Article
Schütz B.
Jurastow I.
Bader S.
Ringer C.
von Engelhardt J.
Chubanov V.
Gudermann T.
Diener M.
Kummer W.
Krasteva-Christ G.
Weihe E.
Chemical coding and chemosensory properties of cholinergic brush cells in the mouse gastrointestinal and biliary tract.
Front. Physiol. 2015; 687https://doi.org/10.3389/fphys.2015.00087
View in Article
Panneck A.R.
Rafiq A.
Schütz B.
Soultanova A.
Deckmann K.
Chubanov V.
Gudermann T.
Weihe E.
Krasteva-Christ G.
Grau V.
et al.
Cholinergic epithelial cell with chemosensory traits in murine thymic medulla.
Cell Tissue Res. 2014; 358: 737-748https://doi.org/10.1007/s00441-014-2002-x
View in Article
Perniss A.
Liu S.
Boonen B.
Keshavarz M.
Ruppert A.-L.
Timm T.
Pfeil U.
Soultanova A.
Kusumakshi S.
Delventhal L.
et al.
Chemosensory Cell-Derived Acetylcholine Drives Tracheal Mucociliary Clearance in Response to Virulence-Associated Formyl Peptides.
Immunity. 2020; 52: 683-699.e11https://doi.org/10.1016/j.immuni.2020.03.005
View in Article
Saunders C.J.
Christensen M.
Finger T.E.
Tizzano M.
Cholinergic neurotransmission links solitary chemosensory cells to nasal inflammation.
Proc. Natl. Acad. Sci. USA. 2014; 111: 6075-6080https://doi.org/10.1073/pnas.1402251111
View in Article
Krasteva G.
Canning B.J.
Papadakis T.
Kummer W.
Cholinergic brush cells in the trachea mediate respiratory responses to quorum sensing molecules.
Life Sci. 2012; 91: 992-996https://doi.org/10.1016/j.lfs.2012.06.014
View in Article
Hollenhorst M.I.
Jurastow I.
Nandigama R.
Appenzeller S.
Li L.
Vogel J.
Wiederhold S.
Althaus M.
Empting M.
Altmüller J.
et al.
Tracheal brush cells release acetylcholine in response to bitter tastants for paracrine and autocrine signaling.
FASEB J. 2020; 34: 316-332https://doi.org/10.1096/fj.201901314RR
View in Article
Martin R.J.
Robertson A.P.
Mode of action of levamisole and pyrantel, anthelmintic resistance, E153 and Q57.
Parasitology. 2007; 134: 1093-1104https://doi.org/10.1017/S0031182007000029
View in Article
Selkirk M.E.
Lazari O.
Hussein A.S.
Matthews J.B.
Nematode acetylcholinesterases are encoded by multiple genes and perform non-overlapping functions.
Chem. Biol. Interact. 2005; 157–158: 263-268https://doi.org/10.1016/j.cbi.2005.10.039
View in Article
Reynolds L.A.
Filbey K.J.
Maizels R.M.
Immunity to the model intestinal helminth parasite Heligmosomoides polygyrus.
Semin. Immunopathol. 2012; 34: 829-846https://doi.org/10.1007/s00281-012-0347-3
View in Article
Drurey C.
Lindholm H.T.
Coakley G.
Poveda M.C.
Löser S.
Doolan R.
Gerbe F.
Jay P.
Harris N.
Oudhoff M.J.
Maizels R.M.
Intestinal epithelial tuft cell induction is negated by a murine helminth and its secreted products.
J. Exp. Med. 2022; 219e20211140https://doi.org/10.1084/jem.20211140
View in Article
Lecomte M.-J.
Bertolus C.
Santamaria J.
Bauchet A.-L.
Herbin M.
Saurini F.
Misawa H.
Maisonobe T.
Pradat P.-F.
Nosten-Bertrand M.
et al.
Selective disruption of acetylcholine synthesis in subsets of motor neurons: a new model of late-onset motor neuron disease.
Neurobiol. Dis. 2014; 65: 102-111https://doi.org/10.1016/j.nbd.2014.01.014
View in Article
El Marjou F.
Janssen K.-P.
Chang B.H.J.
Li M.
Hindie V.
Chan L.
Louvard D.
Chambon P.
Metzger D.
Robine S.
Tissue-specific and inducible Cre-mediated recombination in the gut epithelium.
genesis. 2004; 39: 186-193https://doi.org/10.1002/gene.20042
View in Article
Nadjsombati M.S.
McGinty J.W.
Lyons-Cohen M.R.
Jaffe J.B.
DiPeso L.
Schneider C.
Miller C.N.
Pollack J.L.
Nagana Gowda G.A.
Fontana M.F.
et al.
Detection of Succinate by Intestinal Tuft Cells Triggers a Type 2 Innate Immune Circuit.
Immunity. 2018; 49: 33-41.e7https://doi.org/10.1016/j.immuni.2018.06.016
View in Article
Schneider C.
O’Leary C.E.
von Moltke J.
Liang H.-E.
Ang Q.Y.
Turnbaugh P.J.
Radhakrishnan S.
Pellizzon M.
Ma A.
Locksley R.M.
A Metabolite-Triggered Tuft Cell-ILC2 Circuit Drives Small Intestinal Remodeling.
Cell. 2018; 174: 271-284.e14https://doi.org/10.1016/j.cell.2018.05.014
View in Article
Inclan-Rico J.M.
Siracusa M.C.
First responders: innate immunity to helminths.
Trends Parasitol. 2018; 34: 861-880https://doi.org/10.1016/j.pt.2018.08.007
View in Article
Selkirk M.E.
Lazari O.
Matthews J.B.
Functional genomics of nematode acetylcholinesterases.
Parasitology. 2005; 131: S3-S18https://doi.org/10.1017/S0031182005008206
View in Article
Billipp T.E.
Fung C.
Webeck L.M.
Sargent D.B.
Gologorsky M.B.
McDaniel M.M.
Kasal D.N.
McGinty J.W.
Barrow K.A.
Rich L.M.
et al.
Tuft cell-derived acetylcholine regulates epithelial fluid secretion.
bioRxiv. 2023; (Preprint at)https://doi.org/10.1101/2023.03.17.533208
View in Article
Liu S.
Lu S.
Xu R.
Atzberger A.
Günther S.
Wettschureck N.
Offermanns S.
Members of Bitter Taste Receptor Cluster Tas2r143/Tas2r135/Tas2r126 Are Expressed in the Epithelium of Murine Airways and Other Non-gustatory Tissues.
Front. Physiol. 2017; 8849https://doi.org/10.3389/fphys.2017.00849
View in Article
Bany I.A.
Dong M.-Q.
Koelle M.R.
Genetic and Cellular Basis for Acetylcholine Inhibition of Caenorhabditis elegans Egg-Laying Behavior.
J. Neurosci. 2003; 23: 8060-8069https://doi.org/10.1523/JNEUROSCI.23-22-08060.2003
View in Article
You H.
Liu C.
Du X.
McManus D.P.
Acetylcholinesterase and Nicotinic Acetylcholine Receptors in Schistosomes and Other Parasitic Helminths.
Molecules. 2017; 22: 1550https://doi.org/10.3390/molecules22091550
View in Article
Rosas-Ballina M.
Tracey K.J.
Cholinergic control of inflammation.
J. Intern. Med. 2009; 265: 663-679https://doi.org/10.1111/j.1365-2796.2009.02098.x
View in Article
Darby M.
Schnoeller C.
Vira A.
Culley F.J.
Bobat S.
Logan E.
Kirstein F.
Wess J.
Cunningham A.F.
Brombacher F.
et al.
The M3 Muscarinic Receptor Is Required for Optimal Adaptive Immunity to Helminth and Bacterial Infection.
PLoS Pathog. 2015; 11e1004636https://doi.org/10.1371/journal.ppat.1004636
View in Article
Vaux R.
Schnoeller C.
Berkachy R.
Roberts L.B.
Hagen J.
Gounaris K.
Selkirk M.E.
Modulation of the Immune Response by Nematode Secreted Acetylcholinesterase Revealed by Heterologous Expression in Trypanosoma musculi.
PLoS Pathog. 2016; 12e1005998https://doi.org/10.1371/journal.ppat.1005998
View in Article
Chu C.
Parkhurst C.N.
Zhang W.
Zhou L.
Yano H.
Arifuzzaman M.
Artis D.
The ChAT-acetylcholine pathway promotes group 2 innate lymphoid cell responses and anti-helminth immunity.
Sci. Immunol. 2021; 6eabe3218https://doi.org/10.1126/sciimmunol.abe3218
View in Article
Roberts L.B.
Schnoeller C.
Berkachy R.
Darby M.
Pillaye J.
Oudhoff M.J.
Parmar N.
Mackowiak C.
Sedda D.
Quesniaux V.
et al.
Acetylcholine production by group 2 innate lymphoid cells promotes mucosal immunity to helminths.
Sci. Immunol. 2021; 6eabd0359https://doi.org/10.1126/sciimmunol.abd0359
View in Article
Hepworth M.R.
Daniłowicz-Luebert E.
Rausch S.
Metz M.
Klotz C.
Maurer M.
Hartmann S.
Mast cells orchestrate type 2 immunity to helminths through regulation of tissue-derived cytokines.
Proc. Natl. Acad. Sci. 2012; 109: 6644-6649https://doi.org/10.1073/pnas.1112268109
View in Article
Shimokawa C.
Kanaya T.
Hachisuka M.
Ishiwata K.
Hisaeda H.
Kurashima Y.
Kiyono H.
Yoshimoto T.
Kaisho T.
Ohno H.
Mast Cells Are Crucial for Induction of Group 2 Innate Lymphoid Cells and Clearance of Helminth Infections.
Immunity. 2017; 46: 863-874.e4https://doi.org/10.1016/j.immuni.2017.04.017
View in Article
Alizadeh H.
Murrell K.D.
The intestinal mast cell response to Trichinella spiralis infection in mast cell-deficient w/wv mice.
J. Parasitol. 1984; 70: 767-773
View in Article
González M.I.
Lopes F.
McKay D.M.
Reyes J.L.
Mast cell deficiency in mice results in biomass overgrowth and delayed expulsion of the rat tapeworm Hymenolepis diminuta.
Biosci. Rep. 2018; 38BSR20180687https://doi.org/10.1042/BSR20180687
View in Article
Donaldson L.E.
Schmitt E.
Huntley J.F.
Newlands G.F.
Grencis R.K.
A critical role for stem cell factor and c-kit in host protective immunity to an intestinal helminth.
Int. Immunol. 1996; 8: 559-567https://doi.org/10.1093/intimm/8.4.559
View in Article
Tsai M.
Valent P.
Galli S.J.
KIT as a master regulator of the mast cell lineage.
J. Allergy Clin. Immunol. 2022; 149: 1845-1854https://doi.org/10.1016/j.jaci.2022.04.012
View in Article
Schmitt M.
Schewe M.
Sacchetti A.
Feijtel D.
van de Geer W.S.
Teeuwssen M.
Sleddens H.F.
Joosten R.
van Royen M.E.
van de Werken H.J.G.
et al.
Paneth Cells Respond to Inflammation and Contribute to Tissue Regeneration by Acquiring Stem-like Features through SCF/c-Kit Signaling.
Cell Rep. 2018; 24: 2312-2328.e7https://doi.org/10.1016/j.celrep.2018.07.085
View in Article
Rothenberg M.E.
Nusse Y.
Kalisky T.
Lee J.J.
Dalerba P.
Scheeren F.
Lobo N.
Kulkarni S.
Sim S.
Qian D.
et al.
Identification of a Ckit+ Colonic Crypt Base Secretory Cell that Supports Lgr5+ Stem Cells in Mice.
Gastroenterology. 2012; 142: 1195-1205.e6https://doi.org/10.1053/j.gastro.2012.02.006
View in Article
Haber A.L.
Biton M.
Rogel N.
Herbst R.H.
Shekhar K.
Smillie C.
Burgin G.
Delorey T.M.
Howitt M.R.
Katz Y.
et al.
A single-cell survey of the small intestinal epithelium.
Nature. 2017; 551: 333-339https://doi.org/10.1038/nature24489
View in Article
Turtoi E.
Jeudy J.
Henry S.
Dadi I.
Valette G.
Enjalbal C.
Turtoi A.
Analysis of polar primary metabolites in biological samples using targeted metabolomics and LC-MS.
STAR Protoc. 2023; 4102400https://doi.org/10.1016/j.xpro.2023.102400
View in Article
Gerbe F.
van Es J.H.
Makrini L.
Brulin B.
Mellitzer G.
Robine S.
Romagnolo B.
Shroyer N.F.
Bourgaux J.-F.
Pignodel C.
et al.
Distinct ATOH1 and Neurog3 requirements define tuft cells as a new secretory cell type in the intestinal epithelium.
J. Cell Biol. 2011; 192: 767-780https://doi.org/10.1083/jcb.201010127
View in Article
Article info
Publication history
Published: June 11, 2024
Accepted: April 17, 2024
Received in revised form: November 26, 2023
Received: June 18, 2023
Identification
DOI: https://doi.org/10.1016/j.immuni.2024.04.018
Copyright
© 2024 The Authors. Published by Elsevier Inc.
User license
Creative Commons Attribution (CC BY 4.0) |
How you can reuse
ScienceDirect
Access this article on ScienceDirect
Figures
Graphical Abstract
Figure 1Chat gene expression is restricted to tuft cells in the intestinal epithelium in naive mice and during H. polygyrus infection
Figure 2Permanent and intestinal epithelial cell-restricted recombination driven by the Villin-CreERT2 transgene
Figure 3Tuft cell Chat deficiency delays worm expulsion
Figure 4Chat gene deficiency does not impair establishment of a type 2 immune response
Figure 5Tuft cell-restricted Chat gene deficiency impairs intestinal mastocytosis establishment following H. polygyrus infection
Figure 6Tuft cell-derived ACh is released into the host gut lumen, and ACh directly impairs worm physiology
Figure 7Scheme illustrating the tuft cell effector function during helminth parasite infection
Linked Article
Billipp et al.
Related Articles
RESEARCH JOURNALS
TRENDS REVIEWS JOURNALS
PARTNER JOURNALS
AUTHORS
REVIEWERS
NEWS & EVENTS
MULTIMEDIA
ABOUT
CONTACT
CAREERS
ACCESS
COLLECTIONS
INFORMATION
The content on this site is intended for healthcare professionals and researchers across all fields of science.
We use cookies to help provide and enhance our service and tailor content. To update your cookie settings, please visit the Cookie 設定 for this site.
All content on this site: Copyright © 2024 Elsevier Inc., its licensors, and contributors.
All rights are reserved, including those for text and data mining, AI training, and similar technologies.
For all open access content, the Creative Commons licensing terms apply.
この記事が気に入ったらサポートをしてみませんか?